Druckbare, hochempfindliche flexible Temperatursensoren zur Überwachung der Körpertemperatur des menschlichen Körpers:Ein Rückblick
Zusammenfassung
In den letzten Jahren hat sich die Entwicklung und Erforschung flexibler Sensoren nach und nach vertieft, und auch die Leistung tragbarer, flexibler Geräte zur Überwachung der Körpertemperatur hat sich verbessert. Für den menschlichen Körper spiegeln Änderungen der Körpertemperatur viele Informationen über die menschliche Gesundheit wider, und anormale Änderungen der Körpertemperatur weisen normalerweise auf einen schlechten Gesundheitszustand hin. Obwohl die Körpertemperatur unabhängig von der Umgebung ist, wird die Körperoberflächentemperatur leicht von der Umgebung beeinflusst, was die Geräte zur Überwachung der Körpertemperatur vor Herausforderungen stellt. Um eine empfindliche Echtzeit-Erfassung der Temperatur verschiedener Teile des menschlichen Körpers zu erreichen, haben Forscher viele verschiedene Arten hochempfindlicher flexibler Temperatursensoren entwickelt, die die Funktion der elektronischen Haut perfektioniert und auch viele praktische Anwendungen vorgeschlagen. Dieser Artikel gibt einen Überblick über den aktuellen Forschungsstand hochempfindlicher gemusterter flexibler Temperatursensoren, die zur Überwachung von Körpertemperaturänderungen verwendet werden. Zunächst wurden häufig verwendete Substrate und aktive Materialien für flexible Temperatursensoren zusammengefasst. Zweitens werden gemusterte Herstellungsverfahren und Prozesse von flexiblen Temperatursensoren vorgestellt. Anschließend wird die flexible Temperaturerfassungsleistung umfassend diskutiert, einschließlich Temperaturmessbereich, Empfindlichkeit, Reaktionszeit und Temperaturauflösung. Schließlich wird die Anwendung flexibler Temperatursensoren auf Basis hochempfindlicher Muster demonstriert und die zukünftigen Herausforderungen flexibler Temperatursensoren prognostiziert.
Einführung
Alle Lebensaktivitäten des menschlichen Körpers, die auf dem Stoffwechsel und einer relativ konstanten Körpertemperatur basieren, sind für einen gesunden Stoffwechsel notwendig [1]. Hyperthermie oder Hypothermie beeinflusst die Aktivität von Enzymen im Körper, wodurch der normale Betrieb des menschlichen Stoffwechsels beeinträchtigt wird, was zu Störungen verschiedener Zellen, Gewebe und Organe und in schweren Fällen sogar zum Tod führt. Es kann erkennen, dass die relative Stabilität der Körpertemperatur eine notwendige Bedingung für die Aufrechterhaltung einer stabilen Umgebung im Körper und den regelmäßigen Ablauf von Lebensaktivitäten wie dem Stoffwechsel ist. Für den menschlichen Körper spiegeln Veränderungen der Körpertemperatur viele Informationen über die menschliche Gesundheit wider, und abnormale Veränderungen der Körpertemperatur weisen normalerweise auf einen schlechten Gesundheitszustand hin. Bei der Überwachung der menschlichen Gesundheit [2, 3] ist die Körpertemperatur ein wesentlicher Faktor, der nicht ignoriert werden darf, und eine genaue Überwachung der Körpertemperatur in Echtzeit ist besonders wichtig.
Flexible Temperatursensoren haben sich zu tragbaren, hochempfindlichen, tragbaren, großflächigen, genauen und Echtzeit-Trends entwickelt. Der flexible Temperatursensor nutzt hauptsächlich die elektrische Signaländerung des thermosensitiven Materials aufgrund der Temperaturänderung, um die Echtzeitüberwachung der Temperatur zu realisieren [4]. Es nutzt auch den Charakter des flexiblen Substrats, um streng auf der Haut zu haften, um seine Funktion zu verwirklichen. Im Vergleich zu herkömmlichen Temperaturmessgeräten sind sie nicht nur schwer zu tragen, teuer und für Überwachungszwecke anwendbar, es gibt auch Einschränkungen aufgrund der beabsichtigten oder unbeabsichtigten Bewegungen des Patienten und der Unfähigkeit, bestimmte Stellen (wie Wunden) zu überwachen [5 ], Tumorablationsstellen im Körper [6]), können leicht zu ungenauen oder unvollkommenen Messergebnissen führen. Um die oben genannten Probleme zu lösen, sind tragbare flexible, dünne und empfindliche gemusterte Temperatursensoren zu einem Forschungs-Hotspot für wissenschaftliche Forscher geworden.
In den letzten Jahren hat sich die Forschung an flexiblen Temperatursensoren zur Überwachung der Körpertemperatur kontinuierlich weiterentwickelt und es gibt viele Innovationen [7]. Der Einsatz der strukturierten Fertigung zur großflächigen Fertigung flexibler Temperatursensoren hat sich zu einem Entwicklungstrend entwickelt [8]; Die Nachahmung biologischer Strukturen in der Natur ist eine hervorragende Idee [9]. Die Krakenfüße mit Adsorptionseigenschaften, die Wangen der Viper, die Veränderungen der biologischen Temperatur wahrnehmen können [10], und die schnurrhaarähnlichen Strukturen [11] einiger Gliederfüßer oder Säugetiere haben auch eine Temperatursensorfunktion; Um die vom Sensor überwachte Körpertemperatur klar anzuzeigen, werden die Forscher den flexiblen Temperatursensor angeordnet [12,13,14], und das bildgebende Gerät oder sein elektrochromes Material können die Wärmebildabbildung visualisieren [5, 15 , 16]. Die eingehende Forschung zu flexiblen Temperatursensoren bietet auch entsprechende technische Unterstützung, um hohe Anforderungen wie die hochempfindliche Überwachung der Körpertemperatur zu erfüllen.
Dieser Artikel gibt einen Überblick über den jüngsten Forschungsfortschritt hochempfindlicher flexibler Temperatursensoren bei der Überwachung der Körpertemperatur des menschlichen Körpers, wärmeempfindlichen Materialien, Herstellungsstrategien, grundlegender Leistung und Anwendungen. Der erste Teil wird Materialien für flexible Temperatursensoren auswählen und eine Vielzahl von flexiblen Substraten zusammenfassen, wärmeempfindliche Materialien, die als flexible Temperaturüberwachungssensoren verwendet werden können. Der zweite Teil konzentriert sich auf den Einsatz flexibler Temperatursensoren in der Literatur der letzten Jahre. Das gemusterte Herstellungsverfahren wird überprüft und zeigt den typischen Herstellungsprozess. Der dritte Teil stellt die kritischen Leistungsparameter von Temperatursensoren vor. Der vierte Teil zeigt die Anwendungsszenarien und praktischen Anwendungen flexibler Temperatursensoren in den letzten Jahren. Abschließend werden die potenziellen Herausforderungen und zukünftigen Entwicklungsperspektiven von druckbaren, hochempfindlichen flexiblen Temperatursensoren kurz diskutiert.
Methoden
Material
Flexible Substrate
In den letzten Jahren hat die Anwendung und Erforschung flexibler Materialien in den Bereichen Elektrotechnik sowie Medizin und Gesundheit sukzessive zugenommen. Die Herstellung flexibler Sensoren erfordert, dass der Sensor selbst flexibel, dehnbar und duktil ist und die Substrate und Schaltungen, von denen er abhängt. Spezifische Dehn- und Dehneigenschaften zur Anpassung an die Haftung auf der menschlichen Körperoberfläche, gängige flexible Substrate werden meist zu einer Folie verarbeitet, wie Polydimethylsiloxan (PDMS) [17,18,19,20], Polyimid (PI) [21, 22 ], Polyurethan (PU) [23], Polyethylenterephthalat (PET) [24, 25], Polyvinylalkohol (PVA) [26], Polyvinylbutyral (PVB) [27], Papier [28, 29], Silikonkautschuk [5 , 30, 31], und es können auch hautfreundlichere biologisch abbaubare Materialien verwendet werden, wie Pektin [32], Baumwolle, Seide [33] und andere Zellulosematerialien [34, 35].
Das derzeit am häufigsten verwendete flexible Substrat in flexiblen Temperatursensoren ist PDMS, ein ausgezeichnetes thermisch und elektrisch isolierendes Material mit einer relativen Permittivität von 2,3–2,8 und einem spezifischen Volumenwiderstand von \({1}{\text{.2}). } \times {10}^{{14}} \,\Omega\,{\text{cm}}^{ - 1}\), sein spezifisches Gewicht ist \(1,03\,{\text{kg}}\ ,{\text{m}}^{ - 3}\) bei 25 °C hat PDMS eine bessere thermische Stabilität und die Wärmeleitfähigkeit von PDMS beträgt \(0,15\,{\text{W}}\,{\text {m}}^{ - 1} \,{\text{K}}^{ - 1}\).[36] Die Glasübergangstemperatur beträgt nur 125 °C, der Wärmeausdehnungskoeffizient (CTE) [37] beträgt \(301\,{\text{ppm}}\,^{\circ } {\text{C}} ^{ - 1}\). Und sein Elastizitätsmodul ist \(\approx 3,7\,{\text{MPa}}\) [38], was Dehnung und Dehnung von mehr als 200% ergibt. Aufgrund seiner hervorragenden Dehnung, Dehnbarkeit, thermoelektrischen Eigenschaften [39,40,41], der hohen chemischen Stabilität und der einfachen Handhabung wird PDMS häufiger in Anwendungen wie der elektronischen Haut verwendet [42]. Polyimid (PI)-Material mit ähnlichen Eigenschaften wie PDMS (in Tabelle 1 gezeigt), PI weist eine schlechte Wärmeleitfähigkeit in der Größenordnung von \(0,1\,{\text{W}}\,{\text{m}}^{ - 1} \,{\text{K}}^{ - 1}\) [43,44,45], mit elektrischem Isolationswiderstand \({1}{\text{.5}} \times {10}^ {{{17}}} \, \Omega \,{\text{cm}}^{ - 1}\) [46]. Die relative Dielektrizitätskonstante 3,0–3,6 bei einer höheren Glasübergangstemperatur (360–410 °C) [47] und einem niedrigeren Wärmeausdehnungskoeffizienten (CTE) (\(16\,{\text{ppm}}\,^ {\circ} {\text{C}}^{ - 1}\)). Es ist der Elastizitätsmodul ≈\(2.8\,{\text{GPa}}\) [6, 48]. Polyurethan (PU)-Materialien mit Bioanpassungsfähigkeit, guter Dehnungsduktilität [49], wirtschaftlichem und praktischem Einsatz in Temperatursensoren zur Überwachung der Körpertemperatur des Menschen [50]. Das dünnschichtige flexible Substrat verfügt nicht nur über hervorragende mechanische Eigenschaften, sondern eignet sich aufgrund seiner hervorragenden thermischen Eigenschaften auch für die Anwendungsforschung im Bereich flexibler Temperatursensoren. Wie in Abb. 1 gezeigt. Neben der Verwendung der oben genannten organischen Polymermaterialien für flexible Sensoren sind auch gängige Stoffe oder andere biologisch abbaubare Materialien wie Stoff [51, 52], Seide [53] und Baumwolle [54] weich und verformbar, leicht, wirtschaftlich, atmungsaktiv, komfortabel, langlebig und wiederverwendbar. Weitere Vorteile werden erwartet und als Basismaterial für flexible Temperatursensoren untersucht.
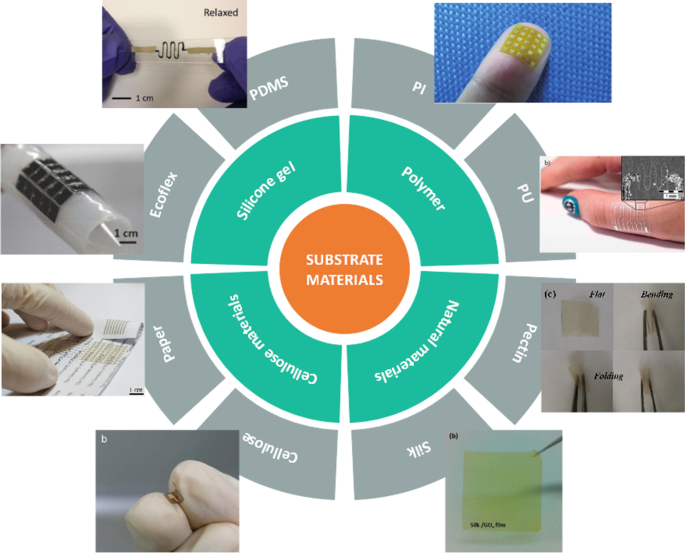
Schematische Darstellung der Substratmaterialien für einen Teil flexibler Sensoren. Im Uhrzeigersinn von rechts oben:Polyimid (PI) [55], Polyurethan (PU) [56], Pektin [32], Seide [33], Zellulose [57], Papier [28], Ecoflex [31] Polydimethylsiloxan (PDMS) [ 58]
Thermisches Material
Das aktive Material ist im Sensor empfindlich, das für die direkte und effektive Reaktion auf Wärmequellen und thermische Signale verantwortlich ist, seine Eigenschaften bestimmen direkt die Leistung des Temperatursensors, einschließlich der Temperaturempfindlichkeit, der Länge der Ansprechzeit, der Haltbarkeit und die Auflösung zur Temperatur [59]. Ein wärmeempfindliches Material, das einfach herzustellen ist, in Rohstoffen vernünftig ist, bioadaptiert ist und ein gewisses Maß an Duktilität und hervorragende Leistung aufweist, ist für die eingehende Forschung zu flexiblen Temperatursensoren attraktiver [60,61,62].
Kohlenstoff
Es wurden große Anstrengungen unternommen, um temperaturempfindliche leitfähige Verbundmaterialien durch Einbringen verschiedener leitfähiger Füllstoffe (wie Materialien auf Kohlenstoffbasis, leitfähige Polymere und Metallpartikel) in Halbleiter- und isolierende Polymermatrizen herzustellen. Gängige Kohlenstoffmaterialien sind Ruß (CB), Graphit (Gr), Kohlenstoffnanoröhren (CNTs) und Graphen.
Ruß (CB) und Graphit werden aufgrund ihrer hervorragenden elektronischen und mechanischen Eigenschaften und der geringen Verarbeitungskosten häufig als leitfähige Füllstoffe verwendet. Unter diesen bildet CB beim Mischen mit Polymeren zu Verbundwerkstoffen leicht Aggregate, und Temperaturänderungen wirken sich auf seine elektrischen Eigenschaften aus [63]. Stabilität führt zu einem höheren Temperaturkoeffizienten des Widerstands (TCR) [64]. Graphit ist ein Allotrop von Kohlenstoff mit guter elektrischer Leitfähigkeit, Wärmeleitfähigkeit und chemischer Stabilität und einem Wärmeausdehnungskoeffizienten von weniger als \(5,0 \times 10^{ - 6} \,{\text{K}}^{ - 1} \) [65]. Im Vergleich zu Ruß ist Graphitpulver als leitfähiger Füllstoff temperaturempfindlicher, und die beiden und ihre Mischungen verringern die Perkolationsschwelle des temperaturempfindlichen Materialverbundfilms, der durch das gefüllte Polymer gebildet wird. Expandierter Graphit (EG) ist ein neuartiger funktioneller Kohlenstoffwerkstoff, der aus natürlichen Graphitflocken durch Einlagerung, Waschen, Trocknen und Hochtemperaturexpansion gewonnene lockere und poröse wurmartige Substanz ist. Die Wärmeleitfähigkeit des durch Heißpressen gebildeten Verbundwerkstoffs kann nach dem Material \(4,70\,{\text{W}}\,{\text{m}}^{ - 1} \,{\text{K}} ^{ - 1} { }\), wenn 10 Gew.-% EG in das PDMS-Präpolymer imprägniert sind [66]. Shih et al. [67]verwendeten PDMS als Basismaterial und Graphitpulver als wärmeempfindliches Sensormaterial und verwendeten den PI-Film durch den Druckprozess, um ein flexibles Temperatursensor-Array aus Gr/PDMS-Verbundsensoren herzustellen (in Abb. 2a gezeigt). Verglichen mit dem klassischen Platin (Pt) Dünnschicht-Temperatursensor (TCR = \({}0,0055\,{\text{K}}^{ - 1}\)) bei gleicher Messung des spezifischen Widerstandes unter Änderung des Umgebungstemperatur (30–110 °C), wenn der Graphitvolumenanteil 15% und 25% beträgt, beträgt der TCR der Verbundmaterialien \(0,286\,{\text{K}}^{ - 1}\) und \ (0.042\,{\text{K}}^{ - 1}\), belegen die Ergebnisse, dass die Empfindlichkeit des Gr-PDMS-Verbundmaterials höher ist. Huang und andere haben ein graphitgefülltes Verbundmaterial aus Polyethylenoxid (PEO) und Polyvinylidenfluorid (PVDF) erforscht und hergestellt [68], (Abb. 2b), das leicht mit variabler Krümmung durch a . an der Oberfläche des menschlichen Körpers befestigt werden kann einfaches Schleuderbeschichtungsverfahren. Ein Temperatursensor mit einem Erfassungsbereich von 25,0–42,0 °C, einer hohen Auflösung von 0,1 °C und hoher Zyklenstabilität, ausgezeichneter Entstörungsfähigkeit (einschließlich Verbiegungsfestigkeit und Wasserdichtheit) und kann den Sensor innerhalb von 1 Monat warten. Es kann die Temperatur der Achselhöhlen über einen langen Zeitraum kontinuierlich messen. Seine hervorragenden mechanischen Eigenschaften können darauf zurückzuführen sein, dass sich die Gr-Partikel als Füllstoffe im Verbund weniger unter verschiedenen Krümmungen bewegen. Die Wärmeleistung hängt mit der Wärmeausdehnung des Polymers zusammen. Als eindimensionales Nanomaterial verbinden sich Kohlenstoffnanoröhren zu einer räumlichen topologischen Struktur [69]. Der Durchmesser beträgt im Allgemeinen 2–20 nm. Es hat viele anormale mechanische Eigenschaften (Elastizitätsmodul bis zu \(1\,{\text{TPa}}\)), elektrische Eigenschaften, einschließlich elektrischer Leitfähigkeit bis zu \(10^{4} \;{\text{S}). }\,{\text{cm}}^{ - 1}\) [70], intrinsische Trägermobilität (\({10.000}\, {\text{cm}}^{2} \,{\text{V }}^{ - 1} \,{\text{S}}^{ - 1}\)) [71]. In den letzten Jahren wurden mit der Vertiefung der Forschung zu Kohlenstoffnanoröhren und Nanomaterialien auch deren breite Anwendungsperspektiven kontinuierlich erschlossen. Kohlenstoffnanoröhren haben eine ausgezeichnete Wärmeübertragungsleistung und CNTs haben ein beträchtliches Seitenverhältnis, sodass sie sich in Längsrichtung befinden. Die Wärmeaustauschleistung ist sehr hoch, und auch die Anwendung flexibler Temperatursensoren wird ständig innovativ, einschließlich hervorragender Designideen und Leistung [72]. Die aus Kohlenstoffnanoröhrchen und Poly(3,4-ethylendioxythiophen)-poly(styrolsulfonat)(PEDOT:PSS) gebildeten Verbundwerkstoffe wurden untersucht, und es wurde festgestellt, dass die Leistung von mehrwandigen Kohlenstoffnanoröhrchen (MWCNTs) und einwandigem Kohlenstoff Nanoröhren (SWCNTs) waren nicht die gleichen [73]. Bei der gleichen Temperatur, die aus MWCNTs und PEDOT:PSS später zusammengesetzten Materialien gebildet wird, verringert der Verbund gleichzeitig die Impedanz des Verbunds, verringert jedoch auch seine Empfindlichkeit gegenüber Temperatur und Feuchtigkeit [74]. Kimet al. [75] verwendeten ein Nassspinnverfahren und verwendeten auch ein PEDOT:PSS-Komposit. Die damit kombinierten SWCNTs können die elektrische Leitfähigkeit und den Leistungsfaktor des Verbundwerkstoffs erheblich verbessern und die Verbundleistung verbessern (Abb. 2d).
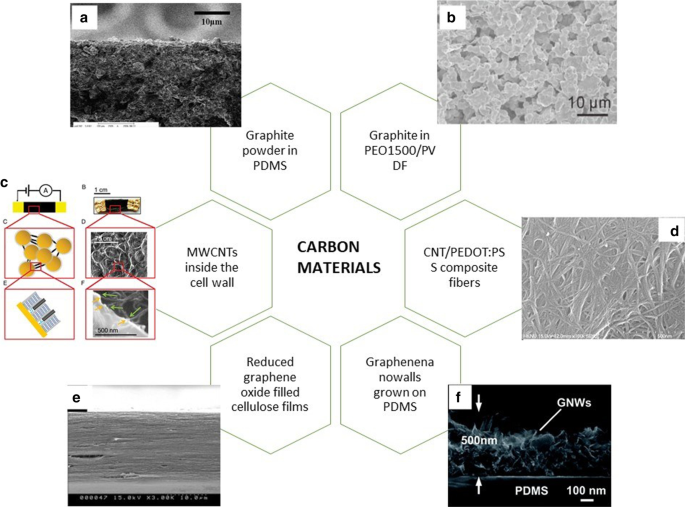
Verschiedene flexible Temperatursensoren auf Basis von Kohlenstoffmaterialien. a REM-Aufnahmen eines Graphit-PDMS-Komposits [67]. b Die ganzflächigen Schliffbilder von PEO1500/PVDF/Gr [68]. c Schematische Darstellungen und rasterelektronenmikroskopische (REM) Aufnahmen von Cyberwood [76]. d REM-Aufnahmen der CNT/PEDOT:PSS-Verbundfasern mit CNT-Gehalten von 40 Gew.-% [75]. e REM-Aufnahmen von CrGO100 [54]. f Das Querschnitts-REM-Bild von GNWs auf PDMS [86]
Herkömmliche Verfahren zur Herstellung biologischer Materialien beruhen auf dem Reverse Engineering biologischer Strukturen, Bionik und biologischer Inspiration. Biologische Strukturen übertreffen oft vom Menschen hergestellte Materialien. Beispielsweise nehmen höhere Pflanzen Temperaturänderungen mit hoher Reaktionsfähigkeit wahr. Gezeigt in Abb. 2c, Giacomo et al. [76] schlugen ein biomimetisches Material mit ausgezeichneter Temperaturempfindlichkeit vor, das aus isolierten Pflanzenzellen und Kohlenstoff-Nanoröhrchen (MWCNT) unter Verwendung von Pflanzen-Nanobiomimetik-Technologie hergestellt wird. Verwendung von CNTs als Kanal, um Zellen direkt zu verbinden, wodurch ein bionisches Material mit einem effektiven Temperaturwiderstand (TCR) von \(- \,1730\% \,{\text{K}}^{ - 1}\) erzeugt wird, das ist zwei Größenordnungen höher als der derzeit beste Sensor und der Überwachungstemperaturbereich beträgt 35–75 °C. Viele Arten der Forschung zu Temperatursensoren können in einem engen Temperaturbereich eine hohe Empfindlichkeit erreichen, aber in einem weiten Temperaturbereich (40 K) ist die Ansprechleistung unzureichend. Siehe auch die biologische Temperaturerfassungsstruktur von Schlangen in der Grubenmembran der Naturschlangen, die biologische Membran hat eine außergewöhnlich hohe Temperatur- und Entfernungsempfindlichkeit. Es kann verwendet werden, um warmblütige Beute in einer bestimmten Entfernung zu lokalisieren. Sie verwendeten die Zugabe von Ca 2+ Ionen in den Pektinfilm, um den Wahrnehmungsmechanismus des Wangenfilms der Schlange nachzuahmen, um ein temperaturempfindliches Pektinhydrogel-Material herzustellen, das mit CaCl2 . versetzt wurde [10]. Es ist möglich, Temperaturquellen mit einer Empfindlichkeit von < 10 mK zu kartieren und zu überwachen und warme Objekte in einer bestimmten Entfernung zu erfassen. Die Kombination von Pflanzenzellen oder die Nachahmung tierischer biologischer Strukturen liefert neue Ideen und erweitert die Forschungsrichtung flexibler Sensoren.
Graphen ist eine zweidimensionale Hybridkohlenstoffschicht mit einem hexagonalen Wabengitter [77, 78]. Es gibt eine große Anzahl delokalisierter Elektronen, wodurch sein einzigartiger Ladungstransport eine hohe Ladungsträgerkonzentration ermöglicht (\({10}^{{{33}}} \,{\text{cm}}^{ - 2}\)), unter bestimmten Umweltbedingungen überschreitet die Mobilität \({10}^{5} \,{\text{cm}}^{2} \,{\text{V}}^{{ - {1}}} \, {\text{s}}^{ - 1}\). Und die räumliche Struktur kann reichlich Bitpunkte bereitstellen. Funktionsgruppen werden modifiziert, um verschiedene Anwendungsanforderungen zu erfüllen. Gleichzeitig hat es eine hohe Fließfähigkeit, ausgezeichnete Wärmeleitfähigkeit, ausgezeichnete Transparenz, mechanische Eigenschaften bis zu \(1\,{\text{TPa}}\) Elastizitätsmodul, chemische Stabilität und Biokompatibilität [79]. Es hat in den Anwendungsgebieten verschiedener elektronischer Geräte viel Aufmerksamkeit auf sich gezogen [80]. Transparentes Graphenoxid (GO) oder reduziertes Graphenoxid (rGO) mit hervorragenden elektronischen und mechanischen Eigenschaften ist ein Produkt mit einer Schichtstruktur, das von Graphitpulver nach Oxidation oder weiterer Reduktion von GO gebildet wird [81, 82]. Die Oberfläche enthält Hydroxylgruppen, Carboxylgruppen und Ringe. Viele funktionelle Gruppen, wie Oxygruppen, werden leicht modifiziert und sind empfindlich gegenüber Umgebungsbedingungen, einschließlich Feuchtigkeit, Temperatur und chemischen Substanzen sowie sichtbaren Reaktionseigenschaften [54, 83]. Die geringe Leitfähigkeit von Graphenoxid (GO) ist jedoch nicht für elektronische Geräte geeignet. Das reduzierte Graphenoxid (rGO) wird durch thermische Reduktion synthetisiert, um die Leitfähigkeit zu verbessern. Seine ausgezeichnete Temperaturempfindlichkeit wird auch für flexible Temperatursensoren benötigt [54, 84, 85]. Graphen-Nanowände (GNWs) werden zu Graphen-Nanoblättern senkrecht zum Substrat gezüchtet, indem eine plasmaunterstützte chemische Gasphasenabscheidung (PECVD) eingesetzt wird [77] und eine polymerunterstützte Transfermethode. Der Wachstumsprozess bildet eine gestaffelte Struktur, um eine höhere Dehnungsleistung zu erreichen [61, 83]. Hervorragende mechanische Eigenschaften haben sich auch für Temperatursensoren in der Forschung bewährt. Die Verwendung von Mehrzweck-Graphen und seinen Derivaten in elektronischen Hautanwendungen ebnet den Weg für flexible Temperatursensoren mit hervorragender Leistung, Transparenz, umfangreichen Funktionen und einem einfachen Herstellungsprozess.
Yanet al. [58] stellten einen flexiblen Temperatursensor her, der dreidimensionales gefaltetes Graphen als aktives Material verwendet und die Temperatur im Bereich von 30–100 °C durch eine lithografische Filtermethode überwachen kann [34]. Aufgrund der Besonderheit der federartigen Struktur kann die Temperaturänderungs-Erfassungscharakteristik bei bis zu 50% Dehnung charakterisieren. Der TCR (\(- \,1,05 \pm 0,28\% \,{\text{K}}^{ - 1}\)) des Sensors unter unbelasteten Bedingungen ist höher als der des berichteten Silizium-Nanodraht-Temperatursensors (\(0,15 - 0,37\% \,{\text{K}}^{ - 1}\)) mal. Es ist erwähnenswert, dass der Wärmeindex des Wärmematerials dieser Struktur eingestellt werden kann. Die Temperaturreaktion und -erholung kann innerhalb von zehn Sekunden abgeschlossen sein, was ihre Anwendbarkeit im Vergleich zu herkömmlichen starren Keramikthermistoren erhöht. Liuet al. [87] verwendeten rGO-Material als temperaturempfindliches Material, um durch Drucktechnologie einen leichten und kostengünstigen, flexiblen Temperatursensor herzustellen. Die Überwachungstemperatur beträgt 20–110 °C, die Empfindlichkeit beträgt \(0,6345\% \,^{ \circ } {\text{C}}^{ - 1}\) und die Reaktionszeit kann 1,2 s erreichen. Mit bestimmten Spannungs- und Dehnungseigenschaften, kann an einer bestimmten Krümmungsfläche befestigt werden. Unter den gleichen experimentellen Bedingungen wurde nach dem Vergleich von reduziertem Graphenoxid (rGO), einwandigen Kohlenstoffnanoröhren (SWCNTs) und mehrwandigen Kohlenstoffnanoröhren (MWCNTs) beim Vergleich von Linearität, Empfindlichkeit, mechanischen Eigenschaften und Wiederholbarkeit festgestellt dass die Leistung des Temperatursensors mit rGO als aktivem Material am ausgewogensten ist, was Ideen für die großflächige Herstellung von elektronischer Haut in der Zukunft liefert. Sadasivuniet al. [54] schlugen eine Verbundfolie vor, die Zellulose als Matrix und thermisch rGO als Füllstoff verwendet, um einen flexiblen und effizienten Überwachungstemperaturbereich von 25–80 °C abhängig von Temperaturänderungen zu erzeugen. (in Abb. 2e gezeigt) Kapazitiver flexibler Temperatursensor mit einer linearen Beziehung. Verglichen mit dem handelsüblichen Platin-Temperatursensor verursacht der Temperatursensor im Laufe der Zeit keine Verschmutzung durch Metallkorrosionsphänomene und kann die Stabilität über lange Zeit aufrechterhalten. Trunget al. [17] stellten einen transparenten und dehnbaren (TS) flexiblen Temperatursensor durch ein einfaches Schleuderbeschichtungsverfahren und eine Laminierungstechnologie her. Die Temperaturerfassungsschicht besteht aus rGO-Nanoblättern und einem elastischen Polyurethan (PU)-Substrat. Verbundmaterialbildung. Die Neuheit des elektronischen Geräts besteht darin, dass jede Materialschicht in der Struktur TS ist und leicht direkt auf ein transparentes und dehnbares Substrat aufgetragen werden kann. Es kann dann einfach am menschlichen Körper befestigt werden. Das Gerät kann Temperaturänderungen von nur 0,2 °C erkennen. Nach 10.000 Dehnungen mit 30% Dehnung hat es fast keinen Einfluss auf das Temperaturverhalten. Das Gerät kann noch verwendet werden, wenn die Belastung 70 % beträgt. Yanget al. [86] schlugen vor, die plasmaunterstützte chemische Gasphasenabscheidung (PECVD) zu verwenden, um eine spezielle verschachtelte 3D-leitfähige GNWs-Netzwerkstruktur auf Kupferfolie zu züchten (Abb. 2f), kombiniert mit einem polymerunterstützten Transferverfahren und PDMS, um ein ultra-empfindliches tragbarer Temperatursensor. Die thermische Reaktion übertrifft die herkömmlicher Temperatursensoren bei weitem. Die ausgezeichnete Duktilität und thermische Empfindlichkeit von GNWs in Kombination mit dem großen Ausdehnungskoeffizienten von PDMS ermöglichen es dem Sensor, die Temperaturänderung von 25–120 °C mit einer Genauigkeit von 0,1 °C, einer Ansprechzeit von 1,6 s und einer Erholungszeit von 8,52 s . zu überwachen , und halten die Stabilität innerhalb von Monaten. TCR erreicht \(0,214\,^{\circ} {\text{C}}^{ - 1}\), was zwei Größenordnungen höher ist als der handelsüblicher Platin-Temperatursensor (\(39,2 \times 10^{ - 4 } \,^{ \circ } {\text{C}}^{ - 1}\)). Die Verwendung von Kohlenstoffmaterialien in flexiblen Temperatursensoren hat ihr Anwendungspotenzial in der Gesundheitsüberwachung, tragbaren Geräten, Robotik, Mensch-Maschine-Schnittstellen und künstlicher Haut gefördert.
Metall und Metalloxid
Metallische Materialien sind im Allgemeinen leitfähige Materialien wie Gold (Au) [88,89,90], Silber (Ag) [91, 92], Kupfer (Cu) [93] und Platin (Pt) [55, 94] (in Abb. 3c), Nickel (Ni) [95] und Aluminium (Al) [96], die hauptsächlich als Elektroden und Drähte von Sensoren verwendet werden. Im Vergleich zum herkömmlichen starren Metalltemperatursensor hat der flexible Metalltemperatursensor eine hohe mechanische Flexibilität, kann leicht an stark gekrümmten Oberflächen angebracht werden und ist besser geeignet, um kleine Temperaturänderungen und -verteilungen in einem kleinen Bereich zu erkennen. Was das aktuelle Druckverfahren betrifft, so sind einige Metallmaterialien temperaturempfindlich und gut leitfähig und bestehen aus leitfähiger Metall-Nanotinte [97], Nanofüller [95], Nanodraht [98] und gemusterter Film zur Herstellung einer aktiven temperaturempfindlichen Schicht [19], die in flexiblen Temperatursensoren weit verbreitet ist.
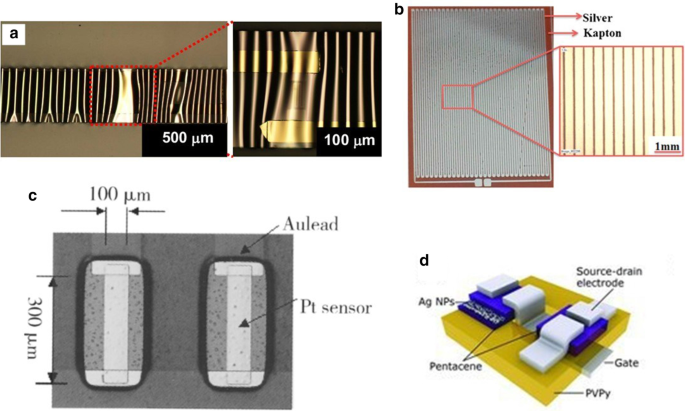
Verschiedene flexible Temperatursensoren auf Basis von Metallmaterialien. a Dehnbare Sensoren auf einem PDMS-Substrat mit periodisch geknickten Mustern [99]. b Fotografien von tintenstrahlgedruckten silbernen Temperatursensoren auf Kapton-Substrat [102]. c Ein Bild der Temperatursensoren [6]. d Die schematische Darstellung des Temperatursensors (ein Transistor)–(ein Thermistor) [101]
Die am häufigsten verwendeten empfindlichen Materialien in typischen Temperatursensoren sind Pt und Au. Binet al. [6] nutzten die Micro-Electro-Mechanical System (MEMS)-Technologie und schlugen Platin als Sensormaterial vor, das nach einem Schälprozess auf dem PI-Film abgeschieden wird. Nachdem das Laminieren das erforderliche Muster gebildet hat, wird es verwendet, um die Temperaturänderung von 20–120 °C zu überwachen, und der TCR-Wert beträgt \(0,0032\,^{ \circ} {\text{C}}^{ - 1}\ ). Im Vergleich zu dem teuren Platin weist Au eine bessere Leitfähigkeit und Flexibilität auf. Um zu erkennen, dass die Leistung des Geräts nicht durch Zugbelastung beeinflusst werden kann, haben Yu et al. [99] nutzten geschickt Sputterabscheidung auf einem vorgestreckten flexiblen PDMS-Substrat aus Chrom(Cr)/Au-Dünnfilm (in Abb. 3a), und ein reversibler bieg- und dehnbarer flexibler Temperatursensor wird durch einen Photolithographieprozess hergestellt. Wenn die maximale Dehnung des Geräts 30 % beträgt, ändert sich die Leistung des Geräts nicht. Die Forschung verbessert den Mangel der schlechten Zugfestigkeit flexibler Sensoren. Dankocoet al. [100] verwendeten eine organische Silberverbundtinte, um durch Tintenstrahldruck glatt und gleichmäßig Silberlinien auf einem Polyimidfilm abzuscheiden (siehe in Abb. 3b). Mit einer messbaren Körperoberflächentemperatur von 20–60 °C lässt sich ein gebogener und flexibler Temperatursensor herstellen. Die durchschnittliche Empfindlichkeit beträgt \(2,23 \times 10^{3} \,^{ \circ } {\text{C}}^{ - 1}\). Der Sensor hat jedoch < 5% Hysterese. Renet al. [101] schlugen einen flexiblen Temperatursensor mit hoher thermischer Auflösung (Dynamikbereich = 10 Bit) basierend auf der Integration von Silbernanopartikeln (NPs)/Pentacen-Thermistor und organischem Dünnschichttransistor (OTFT) mit einem Temperaturbereich von 15–70 ° . vor C. Die Forschung testete die hohe Temperaturabhängigkeit von Verbundmaterialien und bewies die Durchführbarkeit von Silbernanopartikeln (NPs) in Thermistoranwendungen. Sensoren mit hohem Dynamikbereich eignen sich auch für großflächige Sensorarrays und elektronische Haut. Jeonet al. [95] entwickelten einen flexiblen Temperatursensor, der mit einer Mischung aus teilkristallinen Polyethylen (PE) und Polyethylenoxid (PEO) Polymeren, die mit Nickelpartikeln gefüllt sind, konstruiert wurde. Unter diesen kann eine hohe Leitfähigkeit (\(40\,{\text{S}}\,{\text{cm}}^{ - 1}\)) erhalten werden. Mit dem großen positiven Temperaturkoeffizienten (PTC), der für die binäre Polymermischung einzigartig ist, kann der hergestellte Sensor nicht nur einen einstellbaren empfindlichen Temperaturbereich bereitstellen und gleichzeitig die Stabilität des thermischen Zyklus beibehalten, um einen wiederholbaren Temperaturreaktionsprozess zu erreichen. Der Sensor hat eine um drei Größenordnungen höhere Empfindlichkeit (\(0,3\, {\text{V}}\,^{ \circ } {\text{C}}^{ - 1}\)) als Standard-Thermoelemente und drahtlose Übertragung Funktion, aber es gibt einen signifikanten Fehler von ± 3,1 °C. Es ist erwähnenswert, dass sie drahtlose Sensortechnologie mit bedruckbaren Materialien kombinieren möchten, um ein breites Anwendungsspektrum zu erreichen.
Metalloxid ist auch ein wichtiges aktives Material und wird häufig in Temperatursensoren verwendet. Der hohe Temperaturkoeffizient des Widerstands von Metalloxidmaterialien kann die Temperaturerfassungsleistung verbessern. Die thermische Empfindlichkeit von Metalloxid-Halbleitern ist ein Phänomen, bei dem sich die Halbleiterverbindung bei unterschiedlichen Temperaturen ändert und sich der Widerstandswert ändert. Liaoet al. [103] berichteten über einen hochempfindlichen Temperatur-/mechanischen Dual-Parameter-Sensor, der das anorganische thermische Material Vanadiumdioxid (VO2 ), das auf Basis von PET/Vanadiumdioxid im Transferdruckverfahren hergestellt wird (VO2 )/PDMS-Mehrschichtstruktur. Die VO2 Die durch Polymer-unterstützte Abscheidung (PAD) abgeschiedene Schicht wird geätzt und auf dem PDMS-Film befestigt. Es kann die Temperatur im Bereich von 270–320 K erkennen. Die Temperaturerfassungsleistung mit einer Auflösung von 0,1 K ist auf den hohen TCR des VO2 . zurückzuführen Material. Um Veränderungen der Körperoberflächentemperatur genau abzubilden, haben Huang et al. [91] entwickelten ein Tintenstrahldruckverfahren. Verwendung von Nickeloxid (NiO), um eine stabile Nanopartikel-Tinte auf dem PI-Film zu erzeugen. Der winzige quadratische NiO-Film wird an den Enden zwischen die Silberleiterbahnen gedruckt, um Temperatursensoren schnell herzustellen. Es kann die Leistung auch im Biegetest aufrechterhalten und hat eine ähnliche Reaktionsgeschwindigkeit wie ein Thermoelement. Die umfangreiche Forschung zu Metall und Metalloxid hat den Grundstein für die Anwendung von Metallmaterialien im Bereich flexibler Temperatursensoren auf Basis der thermischen Eigenschaften von Metallmaterialien gelegt und interessante Untersuchungen gezeigt.
Polymere und organische Materialien
Polymere sind die am häufigsten verwendeten Materialien in flexiblen Sensoren. Neben der Verwendung als Substrate oder Wirkstoffe sind thermisch empfindliche Verbundmaterialien mit mechanischer Flexibilität, geringem Gewicht, Transparenz, stabiler Leistung, einfacher Verarbeitung und niedrigen Herstellungskosten flexibel. Die Anwendung im Temperatursensor hat viel Aufmerksamkeit erregt. Zu den wärmeempfindlichen Polymeren, die häufig in Temperatursensoren verwendet werden, gehören Poly(3,4-ethylendioxythiophen)-poly(styrolsulfonat) (PEDOT:PSS) [16, 104, 105, 106], Poly(3-hexylthiophen) (P3HT) [107], Polypyrrol ( PPy) [57], pentacene [101], poly (N-isopropyl acrylamide) (pNIPAM) [108], poly(vinylidene fluoride) (PVDF) [109,110,111,112,113], etc.
Based on a circuit design strategy [114] that can improve the accuracy and robustness of a stretchable carbon nanotube temperature sensor, Zhu et al. [115] used differential readout technology to compare the composition of the active, sensitive layer of a stretchable temperature sensor based on OTFTs (see Fig. 4a). Among them, Polystyrene- block-poly(ethylene-ran-butylene)-block-polystyrene (SEBS) with azide-crosslinke and Poly(diketopyrrolopyrrole-[3,2-b]thieno[2′,3′:4,5]thieno[2,3-d]thiophene]) (PDPPFT4) and Poly(isoindigo-bithiophene) (PII2T) these two organic semiconductors (OSCs) are blended and spin-coated on the gate dielectric, CNTs are used as electrodes, and the temperature measurement range is 25–55 °C. Inside, the temperature coefficients of the two sensors are \(- \,2.89\% \,^{ \circ } {\text{C}}^{ - 1}\) and \(- \,4.23\% \,^{ \circ } {\text{C}}^{ - 1}\), respectively. When the uniaxial strain range is 0–30%, the errors are < 1 °C and < 1.5 °C, respectively, further show the feasibility and generalizability of differential readout method and OSCs in stretchable sensors are discussed. Yokota et al. [116] reported a large-area super-flexible temperature sensor based on a semi-crystalline acrylate polymer/graphite composite material that can be measured at multiple points and can be printed (in Fig. 4b). Between 25 °C and 50 °C, it shows noticeable resistance changes at this temperature, which is suitable for measuring the physiological temperature changes of the human body. It has stable thermal cycle stability, the sensitivity of up to 20 mK, and a high-speed response time of less than 100 ms. In in vivo experiments, the stable changes in the rat's lungs' core temperature measured, but the high resolution of the sensor proved to be 0.1 °C. The sensor array based on the above characteristics realizes the dynamic visual, thermal imaging demonstration of the spatial temperature change. However, the air permeability of the equipment components is not right, and the long-term wear is one of the problems to be solved.
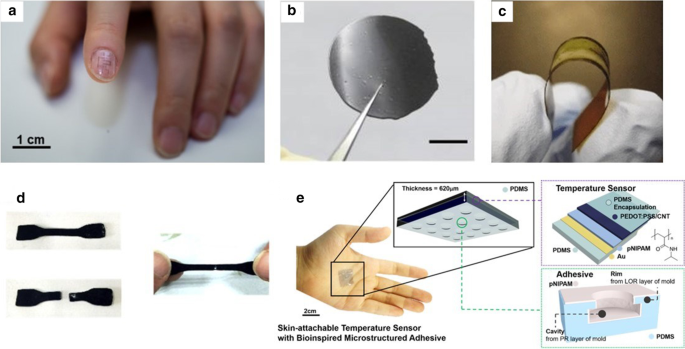
Various flexible temperature sensors based on thermosensitive polymer. a A sample with two temperature sensors on a fingernail [115]. b Photograph of a film of copolymer with graphite filler (scale bar, 1 cm) [116]. c Photograph of a Te-nanowire/P3HT-polymer composite device on a flexible Kapton substrate [107]. d Photos of the DN hydrogels self-healing process [121]. e Photographic image of the fabricated temperature sensor attached onto palm skin and the overall schematic illustration of the octopus-mimicking microstructured adhesive [108]
Self-supplied energy has always been the focus of many people's attention [117]. The realization of self-supplied energy by flexible equipment will significantly reduce the equipment's need for external energy, making flexible equipment more portable and more straightforward [118]. Among them, the thermoelectric polymer materials are the realization of self-supplied energy required. Yanget al. [107]developed a flexible thermoelectric nanogenerator (TENG) based on a clean composite thermoelectric material formed by Te-nanowires grown at room temperature and poly(3-hexyl thiophene) (P3HT) polymer (shown in Fig. 4c). TENG can generate electricity only with a temperature difference of 55 K. Because of the characteristics of thermoelectric materials, TENG can be used as a flexible temperature sensor to monitor the temperature difference of the entire device, and use human body temperature as an energy source to directly power the sensor. The monitoring sensitivity at room temperature is 0.15 K. Besides, they also demonstrated another self-powered temperature sensor with a response time of 0.9 s and a minimum temperature change of 0.4 K at room temperature. The small temperature resolution makes the sensor device can monitor the temperature change of the fingertip. Self-supplied can make flexible sensor equipment more independent and reduce weight. It is also a possibility for the development of flexible sensors in the future (Table 2).
Low-cost, environmentally friendly, easy-to-obtain, and process materials with excellent biocompatibility have always been an essential condition for human beings to pursue to meet mass production continuously [119]. There is such an almost inexhaustible biological material–cellulose, which has excellent properties. Its elasticity and other advantages also play an essential role in flexible sensor devices and can be used as a flexible substrate. Polypyrrole (PPy) is a linear biocompatible polymer with excellent electrochemical stability and rapid response. Mahadeva et al. [57] reported a method based on in-situ polymerization-induced adsorption that combines unique cellulose and nano-thickness polypyrrole (PPy) excellent electrical properties to form a temperature and humidity sensitive composite. The material used to fabricate an environmentally friendly, low-cost, bio-adaptable flexible temperature sensor. Because of the sensitivity of materials to humidity, as the temperature increases, the sensor's capacitance also increases. Hydrogels have received continuous attention in recent years of research [120, 121]. Because of its good self-healing ability, excellent toughness and stretchability, and biological adaptability, it has aroused great research interest in application fields such as flexible electronics, health monitoring, and biomedical diagnosis [122, 123]. However, ionic hydrogel, as a good ion conductor, can respond to a variety of stimuli, hydrogels with weak mechanical strength, and reduced temperature sensitivity present challenges in applying flexible temperature sensors. To solve the disadvantages of traditional hydrogels, An et al. [121] proposed a double-mesh ion-conducting double-network (DN) hydrogel with excellent temperature sensors' self-healing properties. The DN hydrogels self-healing process is shown in Fig. 4d. The addition of carbon nanotubes with high thermal conductivity to the hydrogel with dynamic physical crosslinking and high conductivity hydrophobic association network and ion association network improves the temperature sensitivity of DN hydrogel. The linear hydrogel temperature sensor can perfectly fit the surface of complex objects and produce sensitive resistance changes. The research and development of this material expand hydrogels' application in the fields of biomedicine and flexible electronics.
In recent research, PEDOT:PSS is a new type of organic conductive polymer that often used in printable, flexible temperature sensors [124]. Generally speaking, PEDOT:PSS has the advantages of high conductivity (\(10^{3} \,{\text{S}}\,{\text{cm}}^{ - 1}\)), excellent thermoelectric performance [125,126,127,128], strong stability [123, 129], and transparency when doped [60]. Most polymers are p-type semiconductors. By adding some solvents, such as dimethyl sulfoxide(DMSO) [130] or polyhydroxy organic compounds, such as ethylene glycol [131], the conductivity rate of the polymer can be increased dozens of times or even hundreds of times. Harada and colleagues used different composite ratios of CNTs and conductive PEDOT:PSS solutions to produce a series of composite heat-sensitive films with temperature sensitivity within \(0.25 - 0.78\% \,^{ \circ } {\text{C}}^{ - 1}\) through various printing processes [11, 132,133,134]. The sensing performance is better than the typical metal temperature sensor. Some of the devices exhibited a near body temperature resolution of less than 0.1 °C or fast response time of 90 ms. The research team used various printing methods to create a variety of flexible temperature sensors with different structures and outstanding performance, in addition to enriching the flexible temperature sensors applications in medical and health, wearable devices. Have also triggered everyone thinking about printable, flexible sensors. The fabricating details of printable, flexible temperature sensors will be expanded in the next unit. In addition to changing the composite ratio will affect the performance of the composite film, the structural improvement will also optimize the properties of the same composite film. As Fig. 4e shown, Oh et al. [108]demonstrated a biological material made by a photolithographic stripping process and a spin coating process, inspiringly imitating the adhesion structure of octopus foot sucker. It is a high-sensitivity resistor temperature sensor composed of poly(n-isopropyl acrylamide) (pNIPAM) temperature-sensitive hydrogel, PEDOT:PSS and CNTs. The device has a sensitivity of \(2.6\% \,^{ \circ } {\text{C}}^{ - 1}\) in the temperature range of 25–40 °C, and can accurately detect skin temperature changes of 0.5 °C. Because of the microstructure similar to the suction cup and its viscosity, the device has a certain degree of resistance to bending, non-irritating, long-lasting, and reusable binding effect.
Transparent and scalable nanocomposite field-effect transistor based on polyvinylidene fluoride (PVDF) and its copolymer polyvinylidene fluoride (P (VDF-TrFE)) with high stability, strong mechanical properties, and low distortion, it is often used in pressure sensing, strain sensing, and infrared (IR) light-sensing devices [135, 136]. Interestingly, researchers have found that this type of device is also highly responsive to infrared radiation from the human body, so it is predicted to be used to monitor the human body's physiological temperature changes [137]. Trung and colleagues, based on previous research experience [113, 138], use rGO/(P(VDF-TrFE)) composite sensing active layer as the channel through a simple spin coating process, integrated PEDOT:PSS can fabricate adjustable flexible field-effect transistor (FET) temperature sensor [139]. The film's temperature response and transparency can adjust by changing the concentration of rGO and the thickness of the composite film (Fig. 5a). The sensor can monitor temperature changes from 30 to 80 °C. With 0.1 °C resolution and monitoring ability, and super high-temperature response, excellent temperature sensing performance verifies the feasibility of the application of pyroelectric polymer materials in the field of softcore temperature sensors. Similarly, Tien et al. [112] in order to realize that the sensor can collect pressure and temperature signals without mutual interference, they proposed the use of field-effect transistor (FET) sensing platform to change the material of the response sensitive layer, based on their previous the research concluded that a mixture of polyvinylidene fluoride (P(VDF-TrFE)) and BaTiO3 (BT) nanoparticles (NPs) is used as piezoelectric (see Fig. 5b), pyroelectric gate dielectric and pentacene are used as organic semiconductor channel for pressure thermal resistance directly integrated into the FET platform when the flexible sensor is under multiple stimuli, it decouples the output signal and minimizes the signal interference of strain coupling. The FET sensor can disproportionately present strain and temperature at the same time. The FET sensor array can also visually respond to stimuli, exhibiting the advantages of low energy consumption and low failure, which shows a possibility for the application of large-area multi-modal flexible sensors in the field of electronic skin in the future. Flexible multi-parameter OFET devices that can be printed and fabricated in a large area have excellent application potential in biomedical monitoring, infrared imaging, and electronic skin.
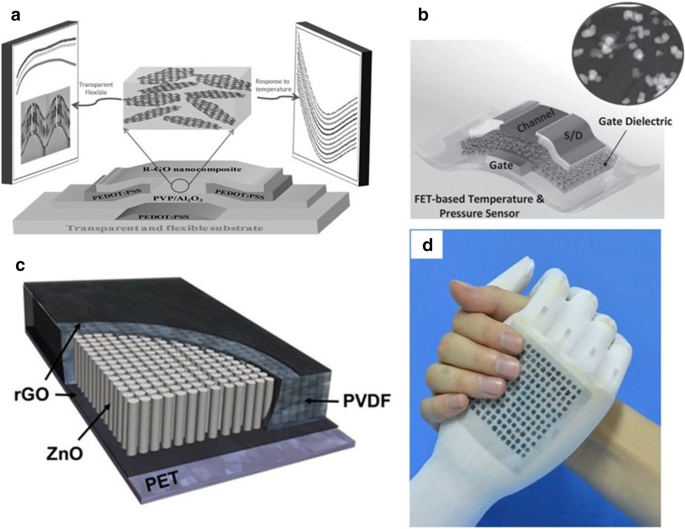
Flexible temperature sensor containing PVDF material. a Schematic of transparent, flexible rGO/P(VDF-TrFE) nanocomposite FET. The schematic illustrates structural, optical (transparent) and electrical (response to temperature) properties of the transparent, flexible R-GO/P(VDF-TrFE) nanocomposite FET [113]. b The structure of physically responsive field-effect transistor (physi-FET) with the bottom-gated and top-contact structure, where the gate dielectric is comprised of nanocomposite of P(VDF-TrFE) and BaTiO3 nanoparticles and the channel is organic semiconductor of pentacene [112]. c Schematic diagram of the ZnO/PVDF composite film and rGO electrodes [110]. d Photograph of the flexible MFSOTE matrixes [23]
In addition to the decoupling method to reduce or eliminate signal interference, in order to solve the problem of mutual interference of multi-parameter flexible sensor signals, Lee et al. [110] proposed a method of inferring temperature based on the recovery time of the resistance change signal, so that the semiconductor zinc oxide (ZnO) nanostructure is mixed into the substrate polyvinylidene fluoride (PVDF) as a filler (As shown in Fig. 5c) to make a highly sensitive multifunctional sensitive layer that can collect temperature and pressure signals at the same time. Among them, the semiconductor ZnO can increase the dielectric constant of PVDF, and it also has thermal stability. Zhanget al. [23] reported a dual-parameter flexible sensor based on a self-powered microstructure-frame-supported organic thermoelectric (MFSOTE) material. The Fig. 5e show the flexible MFSOTE matrixes. By converting the signal changes caused by temperature and pressure stimulation into two independent electrical signals, the temperature and pressure simultaneously sensed. This unique material shows excellent temperature sensing characteristics. The monitoring temperature range is 25–75 °C, The resolution can achieve < 0.1 K, the response time under 1 K temperature difference is < 2 s, and it can also be adjusted according to different substrates to meet the sensing needs.
Fabrication
With the increasing requirements for flexibility, multi-function, simple fabricating, and high sensitivity of electronic devices, the exploration and discovery of flexible sensor fabricating methods with a lightweight, simple process, low cost, and large-area fabricating have always been what researchers are keen [140]. This section mainly summarizes the recently reported and feasible fabricating strategies of flexible temperature sensing elements and discusses the key processes to improve their performance.
Thin Film Deposition
The thin film preparation method can be divided into vapor deposition and phase deposition according to the phase of the material used. The phase deposition includes spin-coating and inkjet printing processes mentioned later. In contrast, the vapor deposition depends on whether the deposition process contains the chemical reaction process divided into physical vapor deposition (PVD) and chemical vapor deposition (CVD).
PVD is to depositions or atoms generated by physical methods on a substrate under vacuum conditions to form a thin film, which generally used to prepare electrodes or active metal layers [141, 142]. Common deposition methods include vacuum evaporation, vacuum sputtering, and ion plating. Among them, the metal target ion sputtering refers to the vacuum container, under the action of high voltage 1500 V, the remaining gas molecules are ionized to form plasma, and the cations bombard the metal target under the acceleration of the electric field, causing the metal atoms to sputter on the surface of the sample to form conductive film [143]. Ahmed et al. [144] introduced a Si-temperature sensor based on a flexible PI substrate. They deposited undoped amorphous silicon as a sensing material between metal electrodes formed by radio frequency magnetron sputtering and packaged them. Finally, the temperature sensing element is embedded in the flexible polyimide film, and the sensing performance is not affected. The maximum TCR at 30 °C is \(0.0288\,{\text{K}}^{ - 1}\). Webb et al. [145] introduced two ultra-thin, skin-like sensor fabricating methods that are self-assembled on the skin surface in the form of an array to provide clear and accurate thermal performance monitoring. A structure is composed of a temperature sensor array, the sensitive layer formed by the serpentine trace structure of the Cr/Au layer deposited on the PI film by the metal evaporation deposition method, the microlithography technology, and the wet etching technology, and the reactive ion etching and metal deposition for contacts and interconnections complete the array. Another sensor structure uses multiplexed addressing to form a patterned PIN diode sensor design of doped Si nano-film. The sensitivity layer is defined by metal evaporation, photolithography, chemical vapor deposition, and wet etching steps. The two arrays are shown in Fig. 6a. Aluminum phthalocyanine chloride (AlPcCl) is often used as a material for solar cells and humidity sensors. Under the study development of Chani et al. [146] AlPcCl is used as a thermistor and deposited on an aluminum electrode on a glass substrate using a vacuum thermal evaporator. The authors found that the AlPcCl film has a higher sensitivity to the temperature at 25–80 °C, and annealing can improve sensing performance. In a flexible temperature sensor developed by Bin et al. [6] that uses Pt resistors as the thermosensitive material, Pt is evaporated on the Al layer deposited on the spin-coated PI film, and the Pt layer is patterned as a sensitive the layer is spin-coated and packaged with polyimide material. After hydrochloric acid treatment, a complete flexible temperature sensor is peeled off, which can be used to measure the surface temperature of objects in the biomedical field.
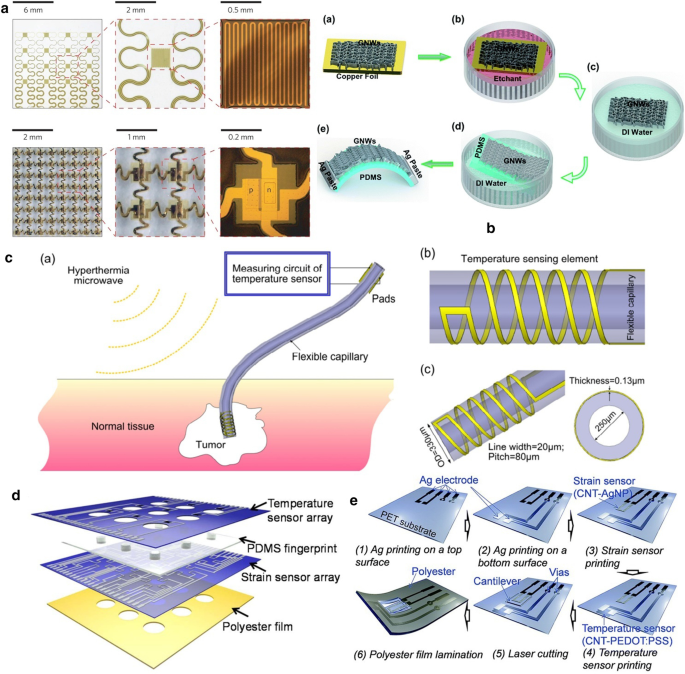
Fabrication method of flexible temperature sensor method of flexible temperature sensor. a Top:Optical images of a 4 × 4 TCR sensor array integrated on a thin elastomeric substrate with magnified views of a single sensor. Bottom:Optical images of a 8 × 8 Si nanomembrane diode sensor array integrated on a thin elastomeric substrate with magnified views of a single sensor [145]. b Schematic process for the fabrication of GNWs/PDMS temperature sensors [86]. c Sketch of the implantable micro temperature sensor on polymer capillary and its application. The head spiral sensing element is fabricated by photolithography [157]. d Schematic for each layer of the e-skin device [132]. e Schematic of the e-skin fabrication process on a PET substrate using a printing method [134]
Compared with other thin-film preparation processes, the chemical vapor deposition method can achieve high-purity and high-quality thin films. It can be structured and controlled at the atomic layer or nanometer level [147,148,149]. The process of synthesizing GNWs film on copper foil by low-pressure radio frequency plasma enhanced chemical vapor deposition (RF-PECVD) technology. Yanget al. [86] developed a flexible temperature sensor based on GNWs/PDMS. The fabricating process is shown in Fig. 6b. They verified GNWs is feasible as an active layer of a temperature sensor, and its thermal response performance exceeds that of a traditional metal temperature sensor. Compared with traditional CVD technology, using PECVD technology [150] under low temperature and low-pressure conditions can effectively improve the deposition rate and film quality. In another study, Zhou et al. used the floating catalyst chemical vapor deposition (FCCVD) method [151] to synthesize the original SWCNT film with a controllable thickness directly. The continuous network of CNTs grown by this method has significant conductivity and a high favorable Seebeck coefficient. After transferring the original SWCNT film to the PET substrate, drop-cast the branched polyetherimide (PEI) ethanol solution, and dry it to obtain an n-type SWCNT film that can be used in the fabricate of flexible thermoelectric modules. Although CVD can achieve the deposition of any material on any substrate, as the demand for simple, low-cost and large-area fabricating nanodevice fabricating technology continues to grow, the fabricating process is complex, high-cost, and toxic CVD growth processes and The time-consuming etching process is being replaced by more suitable flexible electronic device fabricating technology [58].
Micro-nano Patterned Fabrication
Thin-film patterning is one of the core technologies of flexible electronics fabricating. It follows the basic idea of removing materials from top to bottom or adding materials from bottom to top in the fabricating industry. Its key technologies are thin-film fabricating, patterning, transfer, replication, fidelity, and other crafts. Flexible electronics require large-area, low-temperature, low-cost patterning technology. Learned from the patterning technology of microelectronics and micro-electromechanical devices. However, at the same time, we must consider the characteristics of flexible electronic devices such as flexible substrates, organic materials, and large areas. The patterning technologies currently available for flexible temperature sensors include lithography, printing, soft etching, nanoimprinting, inkjet printing, laser sintering, transfer printing, nano-direct writing [152], and other processes.
Lithography is a patterning method to realize various and ingenious geometric figures or structures in flexible electronics. The photolithography process involves transferring the pattern on the photomask to the substrate by using the photoresist with different sensitivity and physical and chemical reactions under the light. The photolithography process usually uses photoresist on an insulator (usually a silicon wafer) to pattern the required pattern or structure after spin coating, and further realize it through a stripping process [153, 154]. Because of the photolithography process and the stripping process, high alignment and etching accuracy, simple mask production, and comfortable process conditions can usually achieve high-precision, feature-rich microstructure systems. In the ultra-thin flexible suture application with an integrated temperature sensor and thermal actuator developed by Kim et al. [155], they used photolithography technology to micro-process the equipment, and the fabricated flexible medical equipment has the stable thermal performance. Lithography technology limited by the necessity of available materials and precision equipment. The thickness of processable materials and thin films are limited. It is not suitable for device fabricating processes that require a large number of active materials. Yanget al. [156] proposed a flexible implantable micro temperature sensor, and used surface microlithography to etch the micro flexible temperature sensor on the outer surface of the polymer capillary (The sensing principle diagram of the miniature thermometer is shown in Fig. 6c). Using Pt as a sensitive material has good linearity, and it has a promising future as an implantable temperature sensor in the biomedical field. However, this technology is the foundation of the microelectronics industry and pioneered the era of wearables flexible electronics.
With the development of science and technology, the printing process has expanded from the traditional text and image field to the micro-nano structure patterning field. technology can deposit various materials on various substrates, and the printing process is not harsh on the environment. In a nutshell, printing technology includes letterpress, lithography, gravure, screen printing, and has evolved into soft etching [158, 159], transfer printing, nanoimprinting, and other methods. According to the specific implementation method, the wearable sensor can distinguish the printed part from the non-printed part with the mask help. In mask printing, the pattern to be transferred must be designed in advance and then formed through the mask. The functional, active material can directly be transferred to the substrate or electrode through the functional ink imprinting process [160]. Screen printing is a typical mask printing technique [161]. In the printing process by absolute pressure, the functional ink is transferred to the substrate through a squeegee with a patterned mesh to form a pattern. The unique printing method allows screen printing to achieve fast, large-area low-cost fabricating requirements on flat or curved surfaces. It has been widely used in fabricating sensor working circuits, electrodes, and sensor sensing elements. Compared with photolithography technology, screen printing can produce patterns on various materials. However, its pattern resolution cannot meet complex geometric shapes requirements and is only suitable for making patterns with simple shapes. Yokota et al. [116] stirred and mixed a variety of semi-crystalline polymers with graphite to form a super-flexible temperature-sensitive copolymer for flexible temperature sensors for human physiological temperature monitoring. The super-flexible temperature sensor element is printed by mask printing by sandwiching the copolymer mixed with graphite filler between two interdigital gold electrodes deposited on the PI film and then forming by hot pressing. Yan et al. [58] used a flat-plate suction filter printing method to deposit graphene through a mask, vacuum filter it, and transfer it to the substrate to form a three-dimensional fold pattern structure produce stretchable graphene with a variable thermal index. The thermistor increases the sensing area and stretchability of the sensor. The pre-designed stretchable sensitive material pattern can still maintain sensitive monitoring of temperature when stretched to less than 50%. To achieve the economical fabricating of sensors with a larger area, Harada and colleagues abandoned complex and costly fabricating processes (such as deposition and photolithography). They chose to fabricate a series of multifunctional flexible sensors using only printing processes. The PEDOT:PSS/CNT composite ink printed on the circuit formed by screen printing on the PET substrate through a shadow mask, and there are holes after laser writing (LS) to combine with the lower layer of PDMS. The fingerprint-like structure (see in Fig. 6d) is combined with the screen-printed strain sensor layer to form a flexible sensor array. The deformation and temperature difference caused by the contact contacts achieve a human-like monitoring performance. In another study, also using full printing technology, Kanao et al. [134] proposed a multifunctional flexible sensor array based on a cantilever beam structure (Fig. 6e). They placed strain sensors and temperature sensors on a flexible screen-printed circuit. On both sides of the PET substrate, a patterned shadow mask with a flexible temperature sensor (PEDOT:PSS/CNT composite ink) printed on the screen's electrical contacts printed circuit. The fully printed array sensor used to imitate the sensing characteristics of human skin. When the cantilever beam structure strained, the heat source is closer to the temperature sensor on the substrate's bottom surface to monitor temperature changes more accurately.
Transfer printing is a printing method that the patterned surface concave structure or convex structure transferred to the receptor substrate through a non-patterned stamp. The basic principle is to use the different viscosity of the printing layer relative to the stamp and the substrate to achieve pattern transfer [162,163,164,165]. There are two types of transfer printing:direct transfer printing and indirect transfer printing. In the fabricate of flexible temperature sensors, the latter often used, that is, the use of a pre-printed patterned film to transfer to the receptor substrate. In the previous review, many examples of organic materials are sensitive layers mentioned, but few inorganic materials are used as temperature-sensitive materials. It is worth noting that Liao et al. [103] reported a high-sensitivity temperature/mechanical dual-parameter sensor containing inorganic thermal material vanadium dioxide (VO2 ), which based on PET/vanadium dioxide fabricated by transfer printing technology (VO2 )/PDMS multilayer structure. The VO2 layer deposited by polymer-assisted deposition (PAD) technology is etched and attached to the PDMS film. After stretching, nano-type spider web cracks formed, and then the layer press to the flexible PET substrate. It can detect the temperature in the range of 270–320 K. The temperature sensing performance with a resolution of 0.1 K attributed to the high TCR of the VO2 Material. The collected temperature signal and the mechanical signal are separated through the algorithm's difference to achieve simultaneous monitoring the effect of temperature and mechanical changes. In order to solve the problem of the flexible temperature sensor's insufficient stretchability, Yu et al. [99], based on transfer printing technology, invented a flexible device that can maintain the sensor performance even when the flexible device is stretched or compressed by 30%. They used the Au/Cr layer as a thermistor to patterned on SOI through standard photolithography technology. The peeled heat-sensitive layer adhered through a flexible PDMS stamp, and then transferred and printed on a pre-stretched flexible PDMS substrate to release the substrate. The fabricating process of forming a stretchable flexible temperature sensor, as shown in the Fig. 7a.
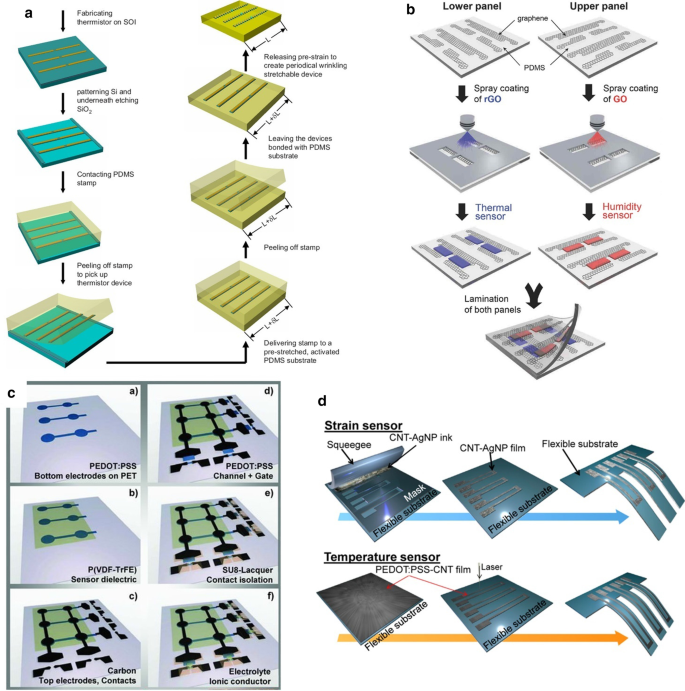
Fabrication method of flexible temperature sensor. a Schematic illustration of the fabrication process [99]. b Fabrication of the stretchable and multimodal all-graphene E-skin sensor matrix [84]. c Process flow illustrating the fabrication of printed ferroelectric active matrix sensor arrays [169]. d Multifunctional e-whisker fabrication [11]
Inkjet printing is an accurate, fast, and reproducible thin-film fabricating technology, which has been widely used in sensor development. Compared with other printing methods, inkjet printing has the advantages of convenience, flexibility, rapidity, low cost, compatibility, accuracy, etc. [166,167,168]. The patterns of inkjet printing need to be post-processed (drying, curing, sintering, etc.) to be fully formed. Improve the performance of printed patterns by converting ink nanoparticles into continuous materials. The properties of the surface tension and viscosity of the ink during the printing process, the quality of the printed pattern also places high requirements on the performance of inkjet equipment [97]. Under the condition of a specific size of the substrate, the conductive track's length formed as long as possible, and the thickness, width, and spacing of the track are reasonable. Repeated experiments obtain the ejection coefficient of the inkjet system. For example, Dankoco et al. [102] used the ink printing method deposit a composite ink with silver as the main component on the PET film to make a flexible and bendable temperature sensor. The circuit on the substrate is clear and smooth, and the ink drops are consistent, which used to measure human body temperature. The picture shows the fabricating process of the extremely sensitive and transparent multifunctional electronic skin sensor matrix developed by Oh et al. [108] The flexible array has the function of monitoring temperature, humidity, and strain. It can feel sensations, such as breathing and touch. GO and rGO, which used as humidity and temperature sensing materials, are sprayed on the PDMS substrate of the graphene circuit grown by the CVD method through inkjet printing technology through a mask. The two sensors are horizontally and vertically aligned, and the temperature sensor is on the bottom layer (as shown in Fig. 7b). After cross-lamination, a PDMS/graphene pressure strain sensor is formed. As a multifunctional flexible sensor, it can collect at the same time but independently respond to a single signal. Inkjet-printed graphene is seven orders of magnitude higher than CVD-grown graphene. The performance advantages reflected in many articles, and some research results are better than CVD-made graphite products. Inkjet printing and screen printing are both rapid and low-cost technical means to realize large-area sensor fabricating. Zirkl et al. [169] combined the two rapid fabricating technologies to create a fully printed flexible sensor array that uses multiple screens. In printing and inkjet printing, only five functional inks used to easily integrate multiple functional electronic components (including pressure and temperature-sensitive sensors, electrochromic displays, and organic transistors) on the same flexible substrate (in Fig. 7c). Because the fabricating speed and low cost of the process can also be applied to the smart sensor network using the roll-to-roll (R2R) fabricating process in the future. The development of a disposable electronic skin system (EES) is particularly critical. Similar to the previous example. Vuorinen et al. [56] introduced a temperature sensor similar to a band-aid after inkjet printing. The sensor uses graphene/PEDOT:PSS composite ink and the printing done on PU material suitable for skin. In particular, in addition to being able to achieve a sensitivity higher than 0.06% \(^{ \circ } {\text{C}}^{ - 1}\), they used inkjet printers to perform serpentine patterned inkjet printing between the silver screen printed with a high resolution (1270 dpi) improved the lack of inkjet printing for printing complex graphics such as snakes. With the use and research of inkjet technology in flexible electronics, the technology for controlling nozzles and inks is also improving. Compared with the speedy fabricating process of screen printing, inkjet printing needs to go through a debugging process, and the printing speed is not as good as screen printing. Also, the small number of nozzles running simultaneously and the high nozzle failure rate limit the inkjet printing fabricating technology to laboratories, and the large-area fabricating requirements of industrial production cannot achieve.
Laser direct writing (LDW) technology uses calculations to design pre-designed patterns. It directly uses laser beam ablation without masking and vacuums deposition. It can directly complete pattern transfer on the surface of the substrate material, with good spatial selectivity and high direct writing speed and processing accuracy, short cycle, high material utilization, and low pollution. Compared with traditional temperature thermistors that require high temperature and a variety of complex processes to activate the sensing function, LDW can achieve selective annealing on a predetermined pattern. A novel integral laser induced by Shin et al. [170] The laser-induced reduction sintering (m-LRS) fabricating scheme can also reduce metal oxide NPs during the annealing process. Scratch NiO NPs ink on a fragile PET substrate, and use m-LRS technology to directly reduce NiO to pattern a linear Ni electrode to form a planar Ni–NiO–Ni heterostructure (as shown in Fig. 8a). The unique method of fabricating a complete flexible temperature sensor system composed of Ni electrodes and NiO sensing channels from a single material NiO NPs provides a new idea for the rapid fabricating of flexible temperature sensors. Different from the previously designed and fabricated fully-printed sensor arrays, Harada et al. [11] proposed a more direct method of mass-fabricating flexible temperature sensors based on previous research. The flexible temperature sensing composite ink printed on the substrate, using the laser for etching away the excess part directly, leaves the designed pattern on the substrate and completes the fabricate of the temperature sensor array. Cut the base to imitate the animal's whiskers to create an artificial electronic whisker (e-whisker) structure (Fig. 7d), strain sensor formed by laminated screen printing. Bionic sensor arrays can scan and perceive three-dimensional objects by using structural advantages.
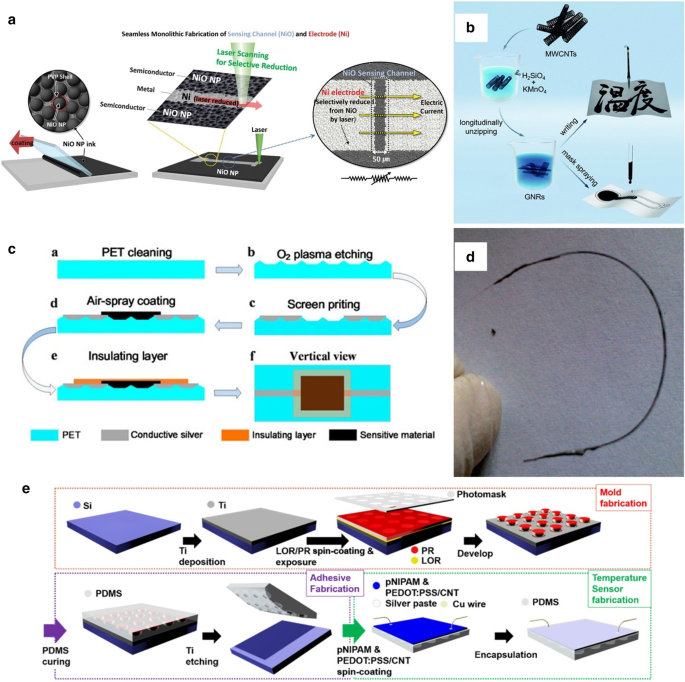
Commonly used fabricating methods. a Schematic of the m-LRS process [170]. b Schematic diagrams showing fabrication process of the paper-based GNRs sensors [171]. c Fabrication process of the temperature sensors [87]. d Flexible temperature sensor on yarn, contacts made of polymer conductive paste [111]. e Fabrication process for the mold, adhesive, and temperature sensor [108]
Under the premise of realizing low-cost and large-area fabricating, disposable intelligent monitoring equipment not only allows monitoring behavior to use anytime and anywhere but also ensures the safety and hygiene of the flexible monitoring equipment. Gong et al. [171] proposed a pen-on-paper fabricating method that uses a brush write or a mask to graphene nanoribbon (GNR) conductive ink dripped between the carbon nanotube electrodes on the paper base. Shown in Fig. 8b. The flexible temperature sensor fabricated after encapsulation with transparent tape. In their research, GNRs have excellent sensing performance and meet the requirements for body temperature monitoring. The fabricating method provides reliable support for the application of fast, large-area disposable flexible equipment.
Other Commonly Used Fabricating Methods
Spin-coating the base layer, active layer, and encapsulation layer by layer is a common way to fabricate flexible temperature sensors by the liquid phase method. Spin coating technology often used for substrate fabricating and packaging protection. For solution-like sensitive materials, the spin coating used. The coating can not only significantly improve production efficiency but also has excellent potential for large-area fabricating. Kimet al. [172] demonstrated the fabricating process of an organic field-effect transistor (OFET) array, using a spin-coating process to design a microstructure on a mold with a crystallized P(VDF-TrFE) material as a gate dielectric and other materials. Material packaging forms OFETs, and the introduction of microstructures enhances the sensing performance. The fabricating process of the bionic structure's [108] flexible temperature sensor fabricated. The adhesive layer of the bionic octopus foot sucker structure is fabricated by a spin coating process, combined with photolithography peeling technology and inverted mold technology (in Fig. 8e). It has an excellent temperature monitoring effect, and the materials used also perform well in terms of human biocompatibility. Liuet al. [87] After surface ionization treatment on the substrate, the flexible electrode was screen-printed, and then the sensitive layer rGO was connected to the flexible wire through the manufacturing method of air-spray coating, and the main structure of the temperature-sensitive flexible sensor was formed after packaging (see in Fig. 8c). It is possible to make robot skins. Huanget al. [68] fabricated a temperature sensor with flexibility, high resolution, and high repeatability in the temperature range of 25–42 °C. They dropped the PEO1500/PVDF/Gr composite solution after stirring and ultrasonic treatment on a polyimide flexible substrate. It was spin-coated at a certain speed to form a layer. It packaged it into a temperature sensor, which verified the medical body temperature transmission sense of possibility and feasibility. Spin-coating technology as an effective fabricating process for wearable devices has also introduced into Wu et al. research [173]. They designed an organic thin-film transistor with heat-sensing ability. Cleverly through the unique three-arm three-dimensional composite polylactide, polylactic acid (tascPLA) solution is spin-coated on the Au gate electrode evaporated on the Si substrate. Then the Au source and drain are deposited on it by a thermal evaporation method. The composite film containing tascPLA also serves as the gate of the OFET dielectric and substrate materials. In flexible electronics, there are not a few that use fabric as the carrier of the sensing element. The dip-coating of conductive yarn/fabric in conductive ink is the most commonly used coating technology for flexible sensors. The development of conductive fabrics provides a reasonable prerequisite for the application of smart fabrics. Sibinski et al. [111] developed a temperature sensor that monitors the temperature range of 30–45 °C. Moreover, it currently realizes filamentary miniaturization on a single yarn. In Fig. 8d shown, the PVDF monofilament fiber is coated with PMMA polymer compound mixed with multi-walled carbon nanotubes (MWCNTs) as a heat-sensitive layer by dip coating technology has good temperature sensitivity and extremely high repeatability. It is used as a fabric easily integrated into knit clothing. Another method, based on the molding properties of organic materials, is also used to fabricate flexible sensor membrane structures or to fabricate samples for testing sensing performance. The fabricating process of the thermoelectric nanogenerator (TENG) studied by Yang et al. [107] The drop-casting film method, after comparison, discards the PEDOT:PSS material, which has a weak drop-casting effect and is easy to break, not only the mixture of Te-nanowires and P3HT polymer in chlorobenzene solution is drop-casted on the flexible Kapton substrate to make a composite film, the composite material is also cast on the white fabric cloth, which also has apparent temperature sensing ability and thermoelectric performance, which can be used in wearable heat collectors. Rich and diverse fabricating methods lay the foundation for the development of flexible electronics. Under the requirements of large-scale and large-scale production, suitable fabricating technology is also continually developing. In the future, perfect wearable flexible devices are expected.
Ergebnisse und Diskussion
Key Parameters of Flexible Temperature Sensor
The flexible temperature sensor can be attached to the human body to monitor the subtle temperature changes on the surface of the human body or real-time temperature monitoring of specific parts, and even the temperature of the core or tissues and organs in the body. They respond to temperature changes by changing the resistance and output the temperature changes as electrical signals. With the increasing demand for flexible electronic devices, the disadvantages of traditional temperature sensors, such as poor scalability, inability to carry, and poor real-time performance, are becoming increasingly unsuitable for flexible wearable devices. Today flexible temperature sensors are required to have high performance, such as high sensitivity, fast response time, reasonable test range, high precision, and high durability to realize the monitoring function better.
Sensitivity
Sensor sensitivity refers to the ratio of the change ∆y of the system response under static conditions to the corresponding input change ∆x , that is, the ratio of the dimensions of output and input. When the sensor output and input dimensions are the same, the sensitivity can be understood as the magnification [21, 174, 175]. The temperature coefficient of resistance TCR (TCR, in \(^{ \circ } {\text{C}}^{ - 1}\)) of the common resistance type flexible temperature sensor is expressed in the following expression, \(\Delta R/R_{0} =s\left( {T - T_{0} } \right)\), is the relative resistance change (\(\Delta R/R_{0}\)) as a function of temperature, where \(s\) represents TCR. If the sensor output and input show a linear relationship, the sensitivity is constant [83]. Otherwise, it will change with the input quantity. Generally speaking, by increasing the sensitivity, higher measurement accuracy can be obtained. Most flexible sensors used for body temperature monitoring only pay attention to temperature changes within 10 °C and therefore require high-temperature sensitivity to capture relatively small temperature changes. Here, several typical methods and concepts for improving the sensitivity of flexible temperature sensors are summarized to provide a favorable reference for further improvements.
The sensing performance of flexible temperature sensors is usually closely related to the properties of materials. One possible way to realize the development of sensitive sensors is to adopt composite materials with visible thermal performance. After temperature stimulation, the internal conductive network will change, which will lead to affect the temperature sensitivity of the device. To improve the temperature sensitivity of the active material, a strategy that converts temperature fluctuations into mechanical deformation is adapted to amplify the response of the conductive network to temperature changes. The thermosensitive material is connected to a substrate with a high positive thermal expansion coefficient to enhance thermal induction deformed. This method has applications in sensors made of graphene, graphite and graphene-nanowalls. For example, in the flexible temperature sensor designed and fabricated by Huang et al. [68], Gr establishes a conductive path in the PEO/PVDF binary composite material. In the process shown in the Fig. 9b. The temperature change will cause the PEO to transform from crystal to amorphous, and melt when the temperature is close to the melting point of PEO, the volume expansion of PEO will destroy the conductive network in the composite material, resulting in a sharp increase in resistivity-increasing the strength of the PTC, which can quickly reaction within a narrow temperature range change. Experiments show that PEO thermal performance plays a leading role in the PTC effect of the device. Another way to improve the sensitivity of a flexible temperature sensor is to use a unique structure. In recent years, to improve temperature sensing performance, various design strategies have been developed by changing the structure. Yuet al. [176] recently proposed a method based on engineering microcrack morphology to change the crack morphology of the PEDOT:PSS film on the PDMS substrate by adjusting the substrate surface roughness, acid treatment time, and pre-stretching degree to improve the temperature sensitivity of the sensor. Figure 9d shows the effect of average crack length and cracks density on temperature sensitivity. The result is that the longer the crack length, the higher the crack density, and the higher the temperature sensitivity. It is proved that the micro-crack structure plays a vital role in the temperature sensitivity of the sensor. It is verified that obtaining a high-density micro-crack structure is the key to obtaining the high-temperature sensitivity of the sensor. High density and high length directly correspond to higher temperature sensitivity. The fabricating process of flexible electronic equipment plays a vital role in the production of the device and can effectively improve the sensitivity of the flexible temperature sensor. Just as Shin et al. [170] used a rapid overall laser-induced reduction sintering (m-LRS) method for fabricating Ni/NiO flexible temperature sensor is different from the inkjet printing method. They directly reduce and sinter the Ni electrode on the NiO layer, and form a high-quality overall contact between the metal electrode (Ni) and the temperature-sensitive material (NiO). Ni–NiO–Ni heterogeneous temperature sensor shows higher temperature sensitivity than other sensors of the same type (Fig. 9e). Raman spectroscopy and X-ray diffraction (XRD) measurements show that the superior sensitivity comes from the unique thermal activation mechanism of the m-LRS process. Besides, since flexible temperature sensors are mostly touch-sensitive, it is inferred that the film's thickness affects the sensitivity. The study of Lee et al. [177] verified the effect of the thickness of the highly sensitive paper base on the sensitivity, they use a simple dipping fabricating method to deposit sensitive materials on the printing paper. Dipping time will affect the thickness of the film. The Fig. 9f also shows the different performance of sensitivity due to different thicknesses. It should be noted that the higher the sensitivity, the narrower the measurement range and, worse, the stability. Therefore, it is necessary to pay attention to increasing stability and accuracy while improving sensitivity.
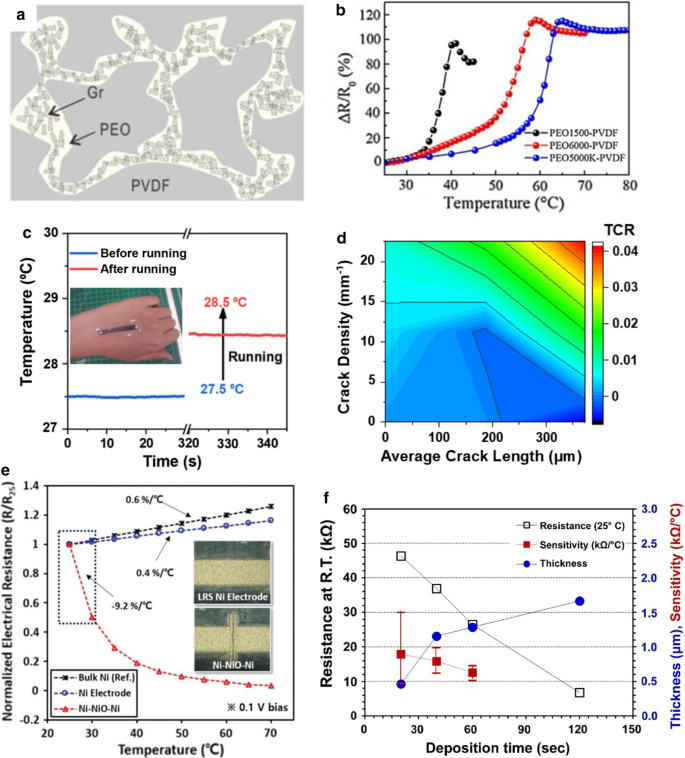
Sensitivity of flexible temperature sensor. a Images of the PEO1500/PVDF/Gr at room temperature. b Resistance curves of PEO1500/PVDF/Gr, PEO6000/PVDF/Gr and PEO5000K/PVDF/Gr composites versus temperature [68]. c The temperature measurement results before and after exercise, the illustration shows the flexible temperature sensor attached to the back of the hand. d Heatmap as functions of average crack length and crack density for TCR valuses from all developed sensors [176]. e PTC and NTC characteristics of the m-LRS processed Ni electrode and Ni–NiO–Ni structure. Inset digital images are m-LRS processed Ni electrode (upper) and Ni–NiO–Ni structure (lower) [170]. f The results of the deposited film thickness (blue circle), resistance at room temperature (white square) and sensitivity (red square) as a function of deposition time [177]
Other Parameters of Flexible Temperature Sensor
The sensing range of a flexible temperature sensor is an important parameter, which refers to the minimum and maximum temperature that can be detected. In this article, we are only interested in the measurement range (30–45 °C) suitable for body temperature monitoring. Another vital performance parameter, response time, is generally defined as the time consumed by the temperature sensor from applying temperature stimulation to generating a stable signal output. Some documents also define the temperature sensor's response time by the time the sensor temperature rises from \(T_{{{\text{sensor}}}}\) to 90% of the temperature rise (\(T_{0}\)) [145]. It is related to the thermal response of the active material itself and reflects the rapid response ability of the temperature sensor to temperature. In terms of applications, such as real-time human body health-monitoring products and wearable artificial intelligent elements with an instant response, all have a shorter response time.
The sensor ability to sense the smallest amount of change to be measured is defined as resolution. In other words, the input quantity starts to change from a non-zero value. At this time, if the input change value does not exceed an absolute value, and the sensor's output does not change, it means that the sensor cannot distinguish the change of the input quantity. Accuracy refers to the ratio of the value of plus or minus three standard deviations near the real value to the range, the maximum difference between the measured value and the real value, and the degree of dispersion of the measured value. For measuring instruments, accuracy is a qualitative concept and generally does not require numerical expression. Because the normal fluctuations of human body temperature within a day are small, high resolution or high accuracy is significant for flexible temperature sensors for body temperature monitoring, determining the broader application of flexible temperature sensors.
Repeatability is the degree of inconsistency of the measurement results for the same excitation quantity when the measurement system performs multiple (more than 3) measurements on the full range in the same direction under the same working conditions. In a flexible temperature sensor, durability means that it maintains a stable sensing function and a complete device capability under a long-term use environment. A flexible temperature sensor with high durability and high repeatability can meet the basic requirements of long-term stable use. Linearity usually defines as the degree of deviation between the actual input–output relationship curve and the ideal fitting curve, usually expressed as a percentage. Therefore, in the linear range, the output signal will be more accurate and reliable. High linearity is also conducive to the input–output signal calibration process and subsequent data optimization processing [58]. The demand for the development of flexible temperature sensors with high linearity is also increasing.
Applications
The ability to live organisms to respond accurately and quickly to external environmental stimuli is an essential feature of life. The induction of temperature allows humans to predict dangers and respond to diseases. Abnormal changes in body temperature often play a crucial reminder and help for early prevention [179]. With the research and development of flexible electronic devices, such as electronic skins, smart health monitoring systems, smart textiles, and biomedical equipment, sensors with the multi-functional signal acquisition are necessary [180, 181]. Among them, the flexible temperature sensor is an indispensable and vital part of medical and health applications [96]. In the past few years, the progress of new materials, new fabricating technologies, and unique sensing methods have provided an essential reference and a solid foundation for the development of a new type of skin-like flexible temperature sensor. In these sensors, some basic performance parameters such as sensitivity, resolution, and response time even exceed natural skin. Although, after a lot of basic and applied research, research results can be transformed from the laboratory to real-world use. However, there are still many challenges waiting to be solved in practical applications. In the following content, we will summarize recent flexible temperature sensors' results in flexible electronics applications with unique, excellent, and practical application examples.
Flexible sensors have the characteristics of large-area deformability, lightweight, and portability, which can realize functions that traditional sensors cannot. Electronic skin integrates various sensors and conductors on a flexible substrate to form a highly flexible and elastic sensor similar to the skin. It converts external stimuli such as pressure, temperature, humidity, and hardness into electrical signals and transmits them to the computer for processing, even can recognize regular objects [14]. An electronic skin with similar functions is a necessary feature of future robots to achieve perception in an unstructured environment. The electronic skin enables the robot to perceive changes in the external environment as sharply as a real person. Although the principle of electronic skin is simple, there are still challenges in covering the surface of the robot with electronic skin. The use of the electronic skin determines, that is, maintaining the device's integrity while maintaining the sensing performance during mechanical deformation. The ideal method of flexible electronic skin is patterned fabricating. Using solution materials, directly deposited on the substrate through printing technology to form patterns, and achieve roll-to-roll (R2R) large-area fabricating under normal temperature and pressure so that the skin has the advantages of considerable size, high yield, low production cost, and environmental protection. Someya et al. [182] have developed an electronic mesh skin with flexibility, large area, integrated temperature, and pressure-sensing capabilities, as shown in the figure. The stretchable electronic skin has multiple heats, and pressure sensors distributed at the nodes and read data through OTFT. Among them, the thermal sensor array is based on organic diodes, prepared on polyethylene naphthalate (PEN) coated with indium tin oxide (ITO) on the surface. The thermal film is mechanically cut by a numerical control cutting machine, and the R2R process prepares the network structure, and then the network film is combined by lamination to complete the preparation of the thermal sensor array. The parylene protective layer is placed on the organic semiconductor layer to act as a flexible gas barrier layer, extending the device's durability from days to weeks and avoiding mechanical damage to transistors and diodes during testing. When the sensor is in its original state, the space is square, and it becomes a rhombus after being stretched, and it can still maintain excellent electrical characteristics when stretched 25% (shown in Fig. 10a). The establishment of the mesh structure expands the use of electronic skin. The distributed structure of multiple parameters and multiple nodes also makes it possible to monitor irregularly shaped objects. A kind of epidermal electronics proposed by Kim et al. [53] refers to ultra-thin flexible electronic devices fixed on the skin's surface (in Fig. 10b). Only through van der waals force can it fit perfectly with the skin and sense the temperature, strain, and dynamic response. Potential applications include physiological state detection (electroencephalogram, electrocardiogram, electromyography), wound detection or treatment, biological/chemical perception, human–computer interaction interface, wireless communication, etcetera. Integrating all devices in the measurement device in a completely different way integrates a variety of functional sensors (such as temperature, strain, electrophysiology), micro-scale light emitting diodes (LEDs), active/passive circuit units (transistors, diodes, resistors), wireless power supply coils, wireless radio frequency communication devices (high-frequency inductors, capacitors, oscillator, and antenna). Skin electronics has the characteristics of ultra-thin, low elastic modulus, lightweight, and ductility. The device is fabricated in the form of winding-shaped filament nanowires or micro-nano thin films, enabling the system to withstand more significant elastic deformation. It can be easily transferred to the skin surface through the soft-touch process, just like a tattoo sticker. Although the electronic skin has wealthy functions, the resonance frequency will drift when the strain exceeds 12%. Besides, the durability of ultra-thin flexible devices also requires attention. The shortcomings mentioned above need to be considered when designing and fabricating future electronic skin systems.
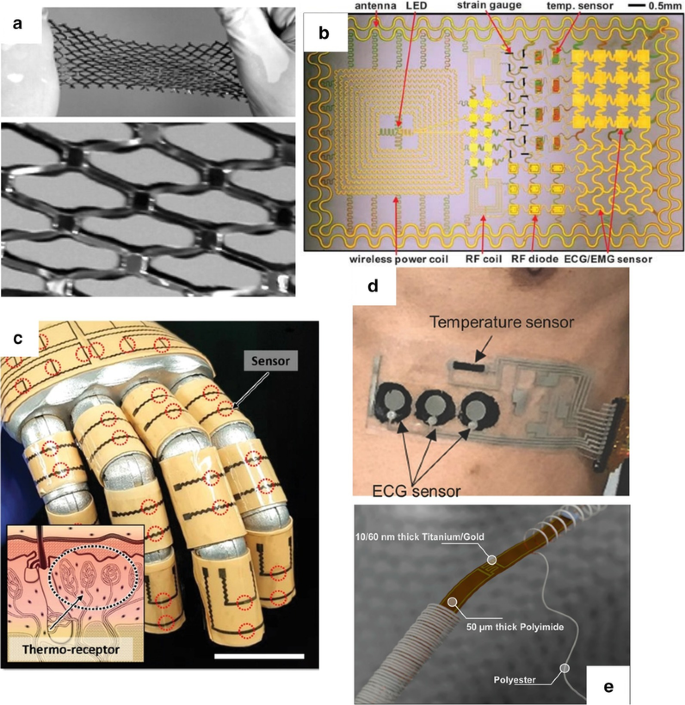
Application demonstration of flexible temperature sensor. a A plastic film with organic transistors and pressure-sensitive rubber is processed mechanically to form a unique net-shaped structure, which makes a film device extendable by 25%. A magnified view of extended net-structures is also shown [187]. b Image of a demonstration platform for multifunctional electronics with physical properties matched to the epidermis [155]. c Model hand covered with the temperature-sensitive artificial skin with enlarged illustration of skin thermos-receptors [170]. d Photo of the fabricated device [184]. e Concept of the flexible temperature sensor embedded within the fibres of a textile yarn [185]
The development of a wearable health monitoring system is for collecting human body thermal parameters:body temperature, epidermal temperature, heat flow [183], etcetera. For observation and inference such as metabolism, fever, disease infection, skin healing, thermal adaptation (implants, prostheses), etc. Compared with the limitations of traditional monitoring systems in the use cases. Flexible wearable temperature monitoring systems have demonstrated more flexible applications and excellent thermal parameter monitoring effects in recent studies—the m-LRS NiO temperature sensor system fabricated by the Shin et al. team that we introduced earlier. In an experiment to verify the potential of its system as an electronic skin application. As shown in the Fig. 10c, three side-by-side temperature sensors attached to the robot finger can make an accurate flow rate of hot or cold water flowing in the microfluidic pipe, flow direction, and temperature response. Besides, it has a high-resolution capability for the temperature increase caused by infrared heat radiation. Moreover, the high-curvature fit feature allows the temperature sensor system also to monitor breathing to provide early warning of abnormal respiratory symptoms caused by disease or poisoning. Experiments on breathing changes before and after exercise have verified the system's effectiveness in monitoring small changes in human breathing and temperature. The holistic fabricating technology they put forward provides useful help for the large-scale development of the healthcare system and electronic skin. Webb et al. [145] reported two types of flexible, ultra-thin, and sensitive temperature sensors/actuators with different structures, namely, a TCR sensor array with a serpentine network formed by Cr/Au fabricated by microlithography technology and a TCR sensor array after deposition. Corrosion nano PIN sensor, the two sensors have high sensitivity, rapid response, and high precision. Non-invasive monitoring of the subtle changes in skin surface temperature caused by changes in human blood flow caused by external stimuli can be realized. By accurately measuring skin thermal conductivity and monitoring skin moisture, it has practical application value for health management such as blood sugar monitoring, drug delivery and absorption, and wound or malignant tumor changes. For a flexible sensor system that needs to monitor health status based on vital signals, easy fabricating, secure attachment, and strong biological adaptability are essential. As Fig. 10d shown, Yamamoto et al. [184] developed a gel-free viscous electrode that is more suitable for electrocardiogram (ECG) sensors. A temperature sensor fabricated by patterned printing is assembled to form a flexible sensor system that can monitor the occurrence of dehydration or heatstroke symptoms. The use of biocompatible materials solves the problem that traditional gels cannot be attached to the human body for a long time. The open, flexible sensing system platform can also add various sensors to develop a broader range of applications. The temperature and heat transfer properties of the skin can be characterized as critical information for clinical medicine and basic research on skin physiology. In terms of thermal adaptation, it is crucial to monitor and predict the residual limb's skin temperature. For example, the prosthesis gaps create a warm and humid micro-environment, which promotes the growth of bacteria and causes inflammation of the skin. Local skin temperature monitoring uses flexible temperature sensors hidden in daily textiles. Due to its high consistency, it is very suitable for non-existent monitoring of skin temperature, which significantly benefits patients and medical staff. Lugoda et al. [185] used different industrial yarn fabricating processes to integrate flexible temperature sensors into fabrics, and studied the sensing effect of embedding flexible temperature sensors in textile yarns. The bending resistance and repeatability of the temperature sensor embedded in the yarn are verified. The elongated sensor is made by patterning a Ti/Au layer deposited on a PI film (see in Fig. 10e). Experiments with the temperature sensor embedded in the armband have verified that this sensor yarn can be used to fabricate intelligent temperature-measuring textile garments. Smart temperature measuring textiles can be used for thermal adaptation monitoring applications such as the detection of thermal discomfort in prostheses, socks for early prediction of diabetic foot ulcers [186], textiles that continuously measure the body temperature of babies, and bandages for monitoring wound infections. The use of industrial yarn fabricating technology to integrate flexible temperature sensors makes large-scale smart textile fabricating possible.
With the development of flexible electronics, the application of multifunctional flexible wearable devices in health care, smart monitoring equipment, human–computer interaction/combination, smart robots, and other related fields have become more and more extensive. Flexible temperature sensors have strong practicability in flexible wearable electronic devices, especially for monitoring human health, which is inseparable from accurate temperature control. The introduction of the wireless transmission function allows people to analyze and utilize the temperature sensor's measurement results. Honda et al. [133] proposed a wearable human–computer interaction device, a patterned "smart bandage" with drug delivery function. The device uses low-cost patterned printing technology to integrate temperature sensors, MEMS-structured capacitive tactile sensors, and wireless coils on a flexible substrate. Figure 11a shows the physical object of the flexible device on the arm. A drug delivery pump (DDP) made by soft lithography and a microchannel for drug discharge can be added to supply drugs to wounds or internal parts to improve the wearer's health. As a wearable interactive humanized health monitoring wireless device application, it will play a positive role in future wearable electronic applications. Intelligent wound patch:wounds after trauma are infected and difficult to heal. Under traditional wound dressings, the healing process of wounds is often unpredictable, and satisfactory repair results cannot be obtained. In wound infection or healing, temperature changes different from the normal body temperature will occur. Therefore, monitoring the subtle temperature changes at the wound is quite necessary for ideal wound healing [188]. To eliminate the "black box" state in the wound healing process and grasp the wound's situation in real-time, Lou et al. [189] proposed a transparent and soft, closed-loop wound healing system to promote wound healing real-time monitoring of the wound. The flexible wound healing system (FWHS) and flexible wound temperature sensing device (FWTSD) likes the form of band-aids, their flexible device in the shape of a double-layered band-aid can be directly attached to the wound. The upper layer is a flexible temperature sensing layer composed of temperature sensors, power management circuits, and data processing circuits. The lower layer is a collagen-chitosan dermis equivalent for skin regeneration. A customized software application (app) installed on the smartphone receives data from the sensing layer, displaying and analyzing wound temperature in real-time (Fig. 11b). The system has high sensitivity and stability, good ductility, reliability, and biocompatibility. In addition to monitoring the normal wound regeneration process, in the biological experiments, timely warning of severe wound infection was achieved. A smart wound healing system with growth promotion, real-time temperature monitoring, wireless transmission, and visualization that integrates wound monitoring, early warning, and on-demand intervention may occur in the future. If the wound after an injury is severely infected and is not treated in time, more serious damage to the body may occur. Timely monitoring of wound conditions and rapid, effective diagnosis and treatment are urgent needs to reduce the occurrence and aggravation of wound infection complications. In another study, Pang et al. [190] proposed a flexible smart bandage with a double-layer structure with high sensitivity, good durability, and remote control. The upper layer is packaged in PDMS connected and integrated through a serpentine wire. The temperature sensor, ultraviolet (UV) light-emitting diode, power supply, and signal processing circuit are designed, and the lower layer is designed with a UV-responsive antibacterial hydrogel. When the temperature sensor detects an abnormal temperature exceeding the threshold, it will wirelessly transmit the signal to the smart device. Moreover, control the in-situ ultraviolet radiation through the terminal application to release antibiotics from the underlying hydrogel and apply it to the wound to achieve early infection diagnosis and processing. The system can monitor wound status in real-time, diagnose accurately, and treat on-demand. The research of this intelligent trauma diagnosis system provides new strategies and diagnostic and treatment ideas for the prevention and treatment of traumatic diseases such as wound treatment and diabetic ulcers. The popularization and application of such systems also have significant prospects. The development of advanced surgical tools is an important measure to improve human health. The advancement of flexible electronics provides reliable support for the flexibility and miniaturization of clinical medical instruments. Simultaneously, the function of flexible devices is enriched, allowing clinical instruments to perform multiple operations on diseased parts in a short period, reducing the risk of surgery. In Fig. 11c,d, Kim et al. [191] invented a direct integration of a multifunctional element group, including a flexible temperature sensor array with the elastic film of a traditional balloon catheter, which provides a variety of functions for clinical applications. The use of this balloon catheter in live animal experiments verified the instrument's ability to provide key information about the depth of the lesion, contact pressure, blood flow or local temperature, and the radiofrequency electrodes on the balloon for tissue controlled local ablation. Specific use in cardiac ablation therapy. In another study, they developed two thin and bendable flexible temperature sensor applications. Used for medical sutures, one of the simplest and most widely used devices in clinical medicine. One is an ultra-thin suture line based on the integrated Au thermal actuator and silicon nanodiode temperature sensor on the biofiber membrane. The other suture line is two \(1 \times 4\) Pt metal temperature sensor arrays formed between Au wires on both sides of the substrate. To achieve the purpose of helping biological wounds heal and monitoring wound recovery. It is full of challenges to build semiconductor devices and sensors and other components on a biologically adaptive platform that touches the flexible curved surface of the human body.
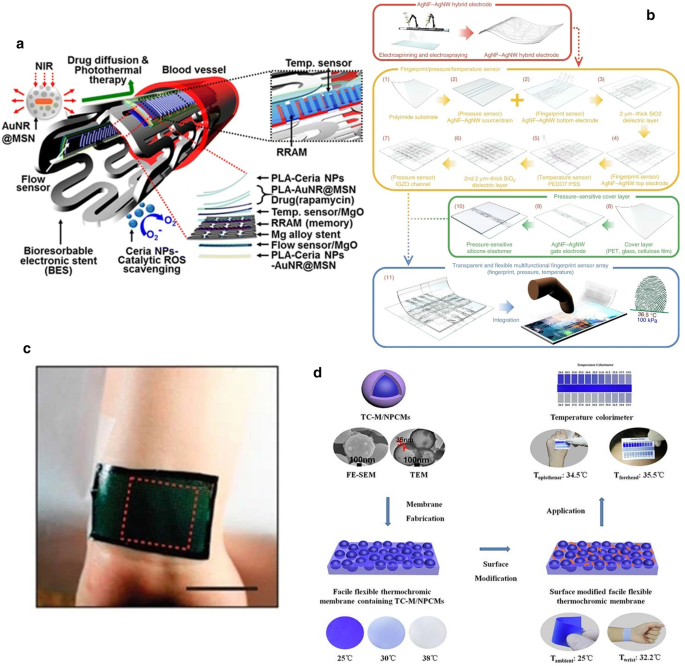
Application demonstration of flexible temperature sensor. a Photos of the smart bandage integrated with touch and temperature sensors, a wireless coil, and a DDP [133]. b The application scenario of FWHS. The FWTSD with a Band-Aid shape tightly contacts with the wound site. Temperature variation is detected by temperature sensor [189]. c Optical image of a multifunctional balloon catheter in deflated and inflated states [191]. d Optimized mechanical structure shown in a schematic illustration when wrapping [53]. e Image of bioresorbable pressure and temperature sensors integrated with dissolvable metal interconnects and wires. f Diagram of a bioresorbable sensor system in the in a rat's skull [193]
As we all know, some operations will leave objects different from the body's tissues such as steel plates, stents, pacemakers, implants, etcetera, inside the body, which will cause discomfort to the body [192]. The development of absorbable devices will optimize surgical implantation equipment Post-access problems reduce the risk of surgery and the difficulty of post-wound healing. Kang et al. [193] reported a multifunctional silicon sensor. The experiment of implanting the sensor in rats' brains proved that all the materials (polylactic-glycolic acid, silicon nanomembranes (Si-NMs), nanoporous silicon, SiO2 ) constituting the sensor could be used. Naturally absorbed through hydrolysis and/or metabolism, no need to extract again. It can continuously monitor parameters such as intracranial pressure and temperature, which illustrates the performance advantages of absorbable devices for treating brain trauma. The emergence of implantable vascular stents provides strong support for the smooth progress of interventional surgery. The vascular stent can expand the blood vessel through a continuous structure in the blocked blood vessel to restore blood flow. However, the inflammation caused by traditional vascular stents in the body for a long time is still difficult to be diagnosed and cured. Son et al. [194] introduced the bioabsorbable electronic stent (BES) applications based on bioabsorbable and bioinert nanomaterials.(Fig. 11e,f) Integrated flow sensing, temperature monitoring, wireless power/data transmission, and inflammation suppression, local drug delivery, and hyperthermia device. The Mg temperature and flow sensor are composed of an adhesion layer, a fiber-shaped Mg resistance (sensing unit), and an outer encapsulation layer (MgO and polylactic acid(PLA)) (as shown in Fig. 12a). Experimental studies have shown that combining bio-absorbable electronic implants and bio-inert therapeutic nanoparticles in intravascular smart stent systems has not yet been recognized.
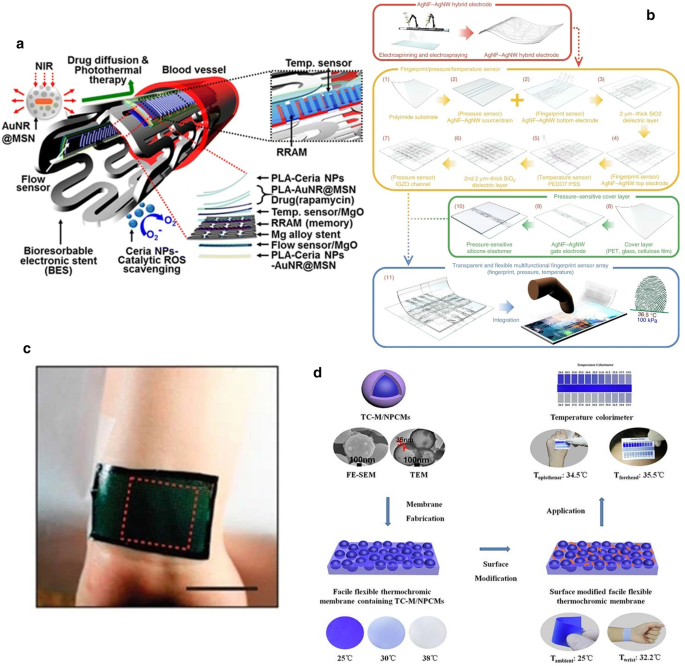
Application demonstration of flexible temperature sensor. a Schematic illustration of the BES (left), its top view (top right), and the layer information (bottom right). The BES includes bioresorbable temperature/flow sensors, memory modules and bioresorbable/bioinert therapeutic nanoparticles [194]. b The fabrication process for the multiplexed fingerprint sensor [196]. c Optical images of an e-TLC device on the wrist during an occlusion test (scale bar, 3 cm) [15]. d Facile flexible reversible thermochromic membranes based on micro/nanoencapsulated phase change materials for wearable temperature sensor [198]
Temperature visualization makes the function of flexible temperature sensors more prominent. It makes up for the disadvantages of traditional infrared imaging equipment that are expensive, unfavorable for carrying, and inaccurate in measuring dynamic objects. The appearance of high-intensity focused ultrasound (HIFU [55]), smart device monitoring, and other occasions will make temperature measurement more accurate and useful [195]. An et al. [196] developed a PEDOT:PSS temperature sensor with ultra-long silver nanofibers (AgNFs) and silver nanowires (AgNWs) hybrid network as a high-performance transparent electrode, layer, and patterned fabricating, using multiplexing technology to create a capacitive flexible and transparent multifunctional sensor array (Fig. 12b). The capacitance change is 17 times higher than that of a pattern sensor that also uses ITO electrodes. In the future, the visual display of fingerprints, pressure, and temperature changes between the fingers on the flexible sensor can be realized simultaneously on smart devices. Applying in the fingerprint recognition function, the security function of smart mobile devices can improve more security. Gao et al. [15] introduced a light-emitting device that combines a colorimetric temperature indicator with wireless, patterned, and stretchable flexible electronic technology. A large-scale thermochromic liquid crystal (TLC) pixel array is formed on a thin elastic substrate in combination with colorimetric reading and radio frequency (RF) drive to map the thermal characteristics of the skin. Under the premise of non-invasiveness, the sensor system uses radio frequency signals to control local heating to survey and map skin temperature with high-temperature accuracy (50 mK) and high spatial resolution. The blood flow under the skin is evaluated by the reactive hyperemia test (see in Fig. 12c). Also, hydration analysis reflects skin health problems. The figure verifies that the device can quickly visually respond to small changes in blood flow. Reflect cardiovascular and skin health problems through hydration analysis. Like the fluoroscopy capability of an infrared camera, the device can be used for core temperature and wound healing monitoring, near-body implant device inspection, malignant tumor cancer screening, and other biomedical-related functions. The unique feature is that the flexible device can be read using a mobile phone, and it can be worn for a long time. The temperature visualization system provides a massive prospect for the description of the thermal properties of the skin. It makes efforts to provide useful indicators for determining the human health and physiological state. In another study, Kim et al. [197] provided a feasible idea using thermochromic materials to observe temperature changes directly. The difference is that their description of the temperature change does not have a specific numerical display, and can only judge the approximate temperature change range. However, as shown in Fig. 12d, He et al. optimized the accuracy of the mapping temperature of thermochromic materials, which can reflect the specific value of the temperature more accurately [198]. In future research, the development of thermochromic materials sensitive to temperature and can accurately respond to temperature will also gradually becoming one research focus. In particular, the reverse cushioning material they made shown a color similar to human skin. Besides, they used 3D printing technology to fabricate the flexible pressure sensor, simplifying the fabricating process, because the sensor structure parameters can be adjusted, showing customizable functions, and achieving the purpose of overall structural fabricating. In the future, 3D printing is the best candidate for the development of unique functional structures [199]. Because this technology can achieve the overall fabricating of the device, it simplifies the fabricating process and ensures integrated molding requirements. It will also gain enormous popularity in the field of flexible sensors.
Schlussfolgerungen
This article reviews the recent research progress of high-sensitivity flexible temperature sensors in human body temperature monitoring, heat-sensitive materials, fabricating strategies, basic performance, and applications. As a relatively stable dynamic variable in the human body, body temperature or local temperature (trauma) may have different degrees of small fluctuations (about 0.5 °C) under the influence of emotions or physiological activities. The monitoring temperature of the flexible temperature sensor needs to be comparable. Traditional infrared cameras have smaller temperature resolution (< 0.1 °C). Besides, timely and fast monitoring of body temperature is another key to breakthrough. The current temperature sensor response time can be within a few milliseconds, but there is a problem of too long reset time. How to shorten the time difference between response time and reset time will directly affect sensor monitoring's efficiency and capability. With the development of multifunctional sensors, how to avoid mutual interference between multiple signals so that the stimuli of the respective responses between the signals can independently respond without mutual interference and accurately output, which has become an urgent problem to be solved and perfected for improving sensor performance. The development of flexible temperature sensors shows us a foreseeable future. In the future, flexible temperature sensors will also achieve large-area low-cost fabricating, high sensitivity, self-supply, visualization, self-healing, biodegradability, and wireless remote sensing transmission [200]. Furthermore, other functions are integrated and put into use. Sorting the collected temperature data into the health big data platform can provide the best help and data support for human future medical diagnosis. Also, patterned micro-nano fabricating technology is a good suggestion for low-cost mass production of sensors. Based on the relatively mature process flow of the printing process, the realization of integrated multifunctional large-area flexible devices is just around the corner. The current flexible temperature sensor can achieve higher sensitivity, but some sensors do not eliminate environmental factors' interference on the temperature sensor. In the future, the flexible temperature sensor used for body temperature monitoring can make efforts to combat environmental influences. Although the flexible sensor itself can be fragile and light, it needs to be connected to the power supply circuit and the power supply, which dramatically reduces the overall flexibility. In the future, for flexible temperature sensors that monitor body temperature, with the further optimization of signal acquisition methods, real-time visual data wireless transmission can be realized under more efficient self-powered conditions, which will be a vast improvement for intelligent monitoring systems. The monitoring of body surface temperature is greatly affected by the environment, while the core temperature is relatively stable. The flexible temperature sensor used for body temperature monitoring can be attached to the body surface (forehead, arm, armpit, etcetera.) to monitor the body surface temperature and even fluctuate. The core temperature with a small range can also be measured. Non-implantable flexible sensors require more improvements in wearability, biocompatibility, and durability to meet the needs of a broader range of people and become a flexible application device available to everyone. For intrusive flexible temperature sensors, whether in the process of intrusion or during the use of the sensor, minimizing damage to the body is the primary consideration. Therefore, exploring and developing biocompatible or biodegradable sensing materials and sensors is undoubtedly an improvement direction. There will be no rejection or allergic reactions in the body due to foreign bodies. In the future, flexible temperature sensors will appear on many occasions around us. The exploration of the development of flexible temperature sensors with high performance, easy fabricating, low cost, and wide application range will continue.
Verfügbarkeit von Daten und Materialien
Nicht zutreffend.
Abkürzungen
- PDMS:
-
Polydimethylsiloxan
- PI:
-
Polyimide
- PU:
-
Polyurethane
- PET:
-
Polyethylenterephthalat
- PVA:
-
Polyvinylalkohol
- PVB:
-
Polyvinyl butyral
- CTE:
-
Coefficient of thermal expansion
- CB:
-
Carbon black
- CNTs:
-
Kohlenstoff-Nanoröhrchen
- TCR:
-
Temperature coefficient of resistance
- ZB:
-
Expanded graphite
- Gr:
-
Graphite
- PEO:
-
Polyethylenoxid
- PVDF:
-
Polyvinylidenfluorid
- PEDOT:
-
PSS:Poly(3,4-ethylene dioxythiophene)-poly(styrene sulfonate)
- MWCNTs:
-
Multi-walled carbon nanotubes
- SWCNTs:
-
Single-walled carbon nanotubes
- GO:
-
Graphenoxid
- rGO:
-
Reduziertes Graphenoxid
- GNWs:
-
Graphene
- PECVD:
-
Plasmaunterstützte chemische Gasphasenabscheidung
- TS:
-
Transparent and stretchable
- Au:
-
Gold
- AG:
-
Silber
- Ku:
-
Kupfer
- Pt:
-
Platinum
- Ni:
-
Nickel
- Al:
-
Aluminium
- MEMS:
-
Micro-electro-mechanical system
- Cr:
-
Chromium
- OTFT:
-
Organic thin-film transistor
- NPs:
-
Nanopartikel
- PE:
-
Polyethylen
- PTC:
-
Positive temperature coefficient
- VO2 :
-
Vanadium dioxide
- PAD:
-
Polymer-assisted deposition
- NiO:
-
Nickel oxide
- P3HT:
-
Poly(3-hexyl thiophene)
- PPy:
-
Polypyrrole
- pNIPAM:
-
Poly (N-isopropyl acrylamide)
- SEBS:
-
Polystyrene- block-poly(ethylene-ran-butylene)-block-polystyrene
- PDPPFT4:
-
Poly(diketopyrrolopyrrole-[3,2-b]thieno[2′,3′:4,5]thieno[2,3-d]thiophene])
- PII2T:
-
Poly(isoindigo-bithiophene)
- OSCs:
-
Organic semiconductors
- TENG:
-
Thermoelectric nanogenerator
- DN:
-
Double-network
- DMSO:
-
Dimethylsulfoxid
- P (VDF-TrFE):
-
Copolymer polyvinylidene fluoride
- IR:
-
Infrarot
- FET:
-
Feldeffekttransistor
- BT:
-
BaTiO3
- ZnO:
-
Zinkoxid
- MFSOTE:
-
Microstructure-frame-supported organic thermoelectric
- PVD:
-
Physikalische Gasphasenabscheidung
- Lebenslauf:
-
Chemische Gasphasenabscheidung
- AlPcCl:
-
Aluminum phthalocyanine chloride
- RF-PECVD:
-
Radio frequency plasma enhanced chemical vapor deposition
- FCCVD:
-
Floating catalyst chemical vapor deposition
- PEI:
-
Polyetherimide
- LS:
-
Laser writing
- R2R:
-
Roll-to-roll
- EES:
-
Electronic skin system
- LDW:
-
Laser direct writing
- GNR:
-
Graphene nanoribbon
- tascPLA:
-
Three-arm three-dimensional composite polylactide, polylactic acid
- XRD:
-
Röntgenbeugung
- m-LRS:
-
Laser-induced reduction sintering
- NTC:
-
Negative temperature coefficient
- ITO:
-
Indium-Zinn-Oxid
- LEDs:
-
Light emitting diodes
- ECG:
-
Electrocardiogram
- DDP:
-
Drug delivery pump
- FWHS:
-
Flexible wound healing system
- FWTSD:
-
Flexible wound temperature sensing device
- UV:
-
Ultraviolett
- Si-NMs:
-
Silicon nanomembranes
- BES:
-
Bioabsorbable electronic stent
- HIFU:
-
High-intensity focused ultrasound
- AgNFs:
-
Silver nanofibers
- AgNWs:
-
Silbernanodrähte
- TLC:
-
Thermochromic liquid crystal
- RF:
-
Funkfrequenz
Nanomaterialien
- Die Gesundheitssensoren von Maxim für ultrakleine Wearables
- Gefahrenüberwachung auf dem neuesten Stand der Technik für Getreidemühlen
- Kombinierte Sensoren zur Überwachung von Durchfluss- und Temperaturfiltern optimieren die Prozesse in der Molkereiindustrie
- DIY:Temperaturüberwachung und -regulierung für HomeBrew
- Goldnanopartikel für Chemosensoren
- Nanodiamanten für magnetische Sensoren
- Tragbarer Gassensor für Gesundheits- und Umweltüberwachung
- Ultrahochleistungsfähige, flexible Ultraviolettsensoren zur Verwendung in Wearables
- Fragen und Antworten:Druckbare flexible Batterien
- Ultradünne und hochempfindliche Dehnungssensoren