Fortschritte bei eisenoxidbasierten Nanostrukturen für Anwendungen in der Energiespeicherung
Zusammenfassung
Die Nachfrage nach grünen und effizienten Energiespeichern im täglichen Leben steigt ständig, was durch die globalen Umwelt- und Energieprobleme verursacht wird. Lithium-Ionen-Batterien (LIBs), eine wichtige Art von Energiespeichern, ziehen viel Aufmerksamkeit auf sich. Als LIBs-Anode wird Graphit verwendet, dessen theoretische Kapazität jedoch gering ist, sodass es erforderlich ist, eine LIBs-Anode mit höherer Kapazität zu entwickeln. In diesem Aufsatz werden Anwendungsstrategien und Forschungsfortschritte neuartiger Eisenoxide und ihrer Komposite als LIBs-Anode in den letzten Jahren zusammengefasst. Hier zählen wir einige typische Synthesemethoden auf, um eine Vielzahl von Nanostrukturen auf Eisenoxidbasis zu erhalten, wie Gasphasenabscheidung, Co-Präzipitation, elektrochemische Verfahren usw. Zur Charakterisierung der Nanostrukturen auf Eisenoxidbasis, insbesondere der in-situ-Röntgenbeugung und 57 Fe-Mössbauer-Spektroskopie werden ausgearbeitet. Darüber hinaus werden die elektrochemischen Anwendungen von eisenoxidbasierten Nanostrukturen und deren Komposite diskutiert und zusammengefasst.
Grafische Zusammenfassung
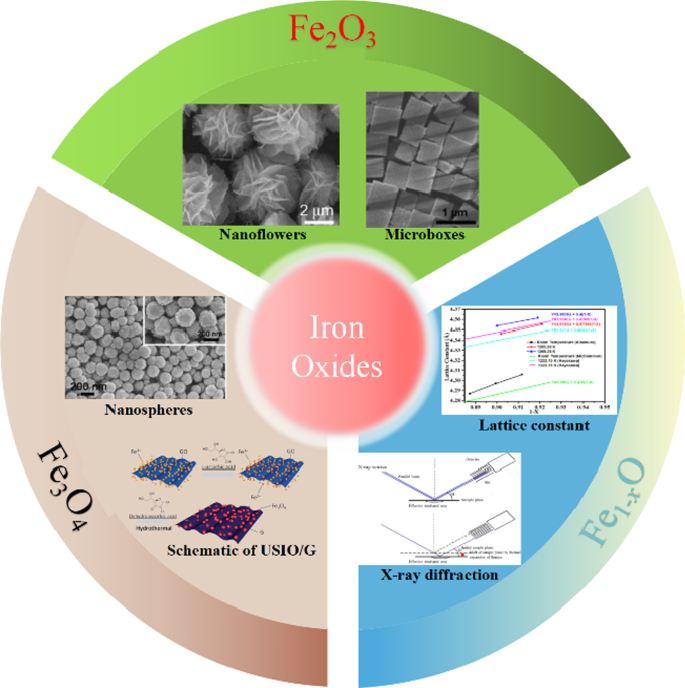
Einführung
Die globalen Energie- und Umweltprobleme führen zu einer steigenden Nachfrage nach hocheffizienter grüner Energie, z. B. Solarenergie, Brennstoffzellen, Lithium-Ionen-Batterien (LIBs) und thermoelektrische Module usw. [1,2,3,4,5,6, 7,8,9,10,11]. Die Forschung und Entwicklung von leistungsstarken und kostengünstigen Energiespeichersystemen ist eine wichtige Lösung für diese Probleme. Unter den Energiespeichergeräten mit breiter Anwendung sind LIBs ein wichtiger Kandidaten für hocheffektive Energiespeichersysteme [12,13,14,15,16,17,18,19,20,21,22,23,24]. Die derzeitige kommerzielle Graphitanode hat jedoch Einschränkungen, z. B. eine relativ niedrige theoretische Kapazität (372 mA h g -1 ) und einige elektrochemisch aktive Materialien werden für LIBs vorgeschlagen [25,26,27,28,29]. Im Jahr 2000 haben Poizot et al. [30] berichteten, dass Übergangsmetalloxide (TMOs) als eine Art wichtige Anoden in LIBs angesehen werden, da ihre theoretischen Kapazitäten 2–3 mal höher sind als die von Graphit. Daher ziehen TMOs und verwandte Verbundstoffe als LIBs-Anode viel Aufmerksamkeit auf sich. Während des Lithium-Insertions-/Extraktionsprozesses haben TMOs die folgende Reaktion [31].
$${\text{M}}_{x} {\text{O}}_{y} + 2y{\text{e}}^{ - } + 2y{\text{Li}}^{ + } \leftrightarrow x{\text{M}}^{0} + y{\text{Li}}_{2} {\text{O}}$$ (1)wobei M für Ni, Cu, Fe, Co usw. steht. Während des Lithium-Insertionsprozesses werden diese Oxide durch Lithium reduziert, und der Verbund besteht aus metallischen Clustern, die in einer Matrix aus amorphem Li2 . dispergiert sind O wird gebildet [30, 31].
Unter den TMOs sind Anoden auf Eisenoxidbasis eine Art ausgezeichnete Kandidaten mit großem Potenzial für LIBs, da sie solche Vorteile besitzen, z. B. Häufigkeit, geringe Kosten und Ungiftigkeit [32, 33]. Jedoch haben Eisenoxide, die als LIBs-Anode dienen, ähnlich wie andere TMOs, zwei kritische Probleme. Eine davon ist die große irreversible Kapazität, die durch die Zersetzung des Elektrolyten und die Bildung einer Festelektrolytgrenzflächenschicht (SEI) im ersten Entladungsprozess verursacht wird. Außerdem wird die Bildung von Fe und Li2 O ist thermodynamisch machbar und die Extraktion von Lithiumionen (Li + ) von Li2 O ist thermodynamisch instabil [34], da ein Teil von Li + kann nicht aus Li2 . extrahiert werden O bildete sich im 1. Entladevorgang. Dies führt auch zu einer teilweise irreversiblen Kapazität. Ein weiteres Problem ist ihre geringe Zyklenstabilität, die hauptsächlich auf eine große Volumenvariation und eine starke Aggregation von Fe bei der Insertion/Deinsertion von Li + . zurückzuführen ist , was zu einer Pulverisierung der Elektroden und einem schnellen Kapazitätsabfall führt [3]. Zur Lösung dieser Probleme werden große Anstrengungen unternommen, um diese Probleme zu überwinden, und es werden einige hochwirksame Ansätze vorgeschlagen. Eine hocheffektive Strategie ist unseres Wissens nach die Nanostrukturierung von Eisenoxiden [3, 35]. Bei einigen einzigartigen Nanostrukturen resultierten die Dehnungs- und Volumenvariationen aus der Einfügung/Deeinfügung von Li + wird weitgehend gehemmt, auch das Li + können leicht in Elektroden diffundiert werden, was zu einer deutlich verbesserten elektrochemischen Leistung der Anode führt [11]. Darüber hinaus werden kohlenstoffhaltige Materialien, z. B. Kohlenstofffasern (CFs), Kohlenstoffnanoröhren (CNTs), Graphen und pyrolysierter Kohlenstoff usw. für die Zusammensetzung mit Eisenoxiden eingeführt [36,37,38]. Die Volumenvariation von Kompositelektroden beim Laden/Entladen kann durch diese kohlenstoffhaltigen Materialien mit einzigartigen Strukturen gepuffert werden, wodurch der elektronische Kontakt und die Zyklenstabilität von Eisenoxid-Nanostrukturen erhöht werden.
In dieser Übersicht werden die kürzlich entwickelten Strategien und wichtige Forschungsergebnisse zu den Eisenoxiden (Fe1−x O, Fe2 O3 , Fe3 O4 ) basierte Nanostrukturen mit Anwendungen in LIBs und Superkondensatoren werden ausgearbeitet und zusammengefasst. Insbesondere konzentrierten wir uns auf die Synthese und das Design der auf Eisenoxiden basierenden Nanostruktur sowie auf deren elektrochemische Leistung.
Wustit (Fe1−x O)
Wustit (Fe1−x O) ist eine nichtstöchiometrische Verbindung mit 0 < x < 0,0464 [11]. Es hat die kubische Struktur aus Steinsalz und seine Gitterkonstante beträgt ~ 4.330 Å [11]. Fe1−x O, verglichen mit Fe2 O3 und Fe3 O4 , hat weniger Anwendungen in der Energiespeicherung, da seine relativ niedrige spezifische Kapazität und metastabile Phase unter 843,15 K, die dazu neigt, sich in Fe und Fe3 zu zersetzen, O4 . Jedoch Fe1−x O, eine vielversprechende Anode für LIBs, hat eine höhere elektrische Leitfähigkeit als die von Fe2 O3 und Fe3 O4 .
Synthese und Charakterisierung
Soweit uns bekannt ist, hat die Zusammensetzung von TMOs mit Hochleistungsabdeckung großes Potenzial für Hochleistungs-LIBs-Anoden [7]. In LIBs wird der elektrochemische Mechanismus der Wustit-Anode als die folgende Gleichung beschrieben [8].
$${\text{FeO}} + 2{\text{Li}}^{ + } + 2{\text{e}}^{ - } \leftrightarrow {\text{Fe}} + {\text{Li }}_{2} {\text{O }}$$ (2)FeO/C-Komposite wurden mit einer einfachen Methode von Gao et al. synthetisiert. [31]. Bei ihrer Synthese wird α-Fe2 O3 Partikel mit einer Größe von 30–120 nm wurden mit Acetylenruß (AB) in verschiedenen Prozentsätzen vermischt und durch Kugelmahlen wurde eine einheitliche Mischung erhalten. Dann wird die Mischung aus α-Fe2 O3 und AB wurde bei 800 °C für 10 h in N2 . kohlenstoffthermisch reduziert Atmosphäre, um einheitliche FeO/C-Verbundstoffe zu erhalten. Der FeO/C-Verbundstoff besitzt eine viel höhere Zyklenstabilität als der von Fe2 O3 /AB-Mischung. Wenn der AB-Gehalt 50 Gew.-% beträgt, beträgt die Kapazität des FeO/C-Verbundstoffs 511 mAh g –1 , höher als 396 mA h g −1 von Fe2 O3 /AB. Außerdem ist die Kapazitätserhaltung nach 50 Zyklen > 96%, offensichtlich 70–80% höher als die von Fe2 O3 /AB. Es ist glaubhaft, dass die überlegene elektrochemische Leistung des FeO/C-Verbundwerkstoffs auf seine höhere elektrische Leitfähigkeit zurückzuführen ist, die auf eine verstärkte Verbindung der FeO- und AB-Partikel nach der kohlenstoffthermischen Reduktion zurückzuführen ist.
Danach, im Jahr 2016, haben Jung et al. [11] stellten ein Kalium(K)-FeO/Graphen-Komposit als LIBs-Anode basierend auf K-dotierten FeO-Nanopartikeln durch thermische Diffusion von K in Fe2 . her O3 /Graphen durch Polyolreduktion. Rhomboedrisches Fe2 O3 Kristalle wurden durch Kalzinieren von K-dotiertem Fe2 . in FeO-Kristalle (kubisch flächenzentriert, FCC) umgewandelt, die einen breiten d-Abstand (5,2 Å) von (111)-Kristallebenen zeigten O3 /Graphen. Vergleich mit zuvor untersuchtem Fe2 O3 /Graphen-Komposit [11], das K-FeO/Graphen zeigte eine Entladekapazität von 1776 mA h g −1 mit hoher Zyklenfestigkeit während 50 Zyklen bei einer Stromdichte von 100 mA g −1 , während Fe2 O3 /Graphen lieferte eine Entladekapazität von 1569 mA h g −1 . Selbst bei einer hohen Stromdichte von 18,56 A g −1 , blieb die Kapazität von K-FeO/Graphen bei 851 mAh g −1 nach 800 Zyklen. Dieser Unterschied ist viel größer, nachdem die Elektroden länger bei einer hohen Stromdichte von 18,56 A g −1 . zykliert wurden . Wie in Abb. 1 gezeigt, verglichen mit dem Fe2 O3 /Graphen hat die K-FeO/Graphen-Anode eine einzigartige Kristallstruktur und einen einzigartigen Reaktionsmechanismus. Die hohe Entladekapazität von K-FeO/Graphen zeigt diese spezifische Kapazität durch Speicherung von zusätzlichem Li + sollte von den Leerstellen und dem breiten d-Abstand innerhalb der Wustit-Gitter durch Kaliumdiffusion in Fe2 . beigetragen werden O3 Gitter.
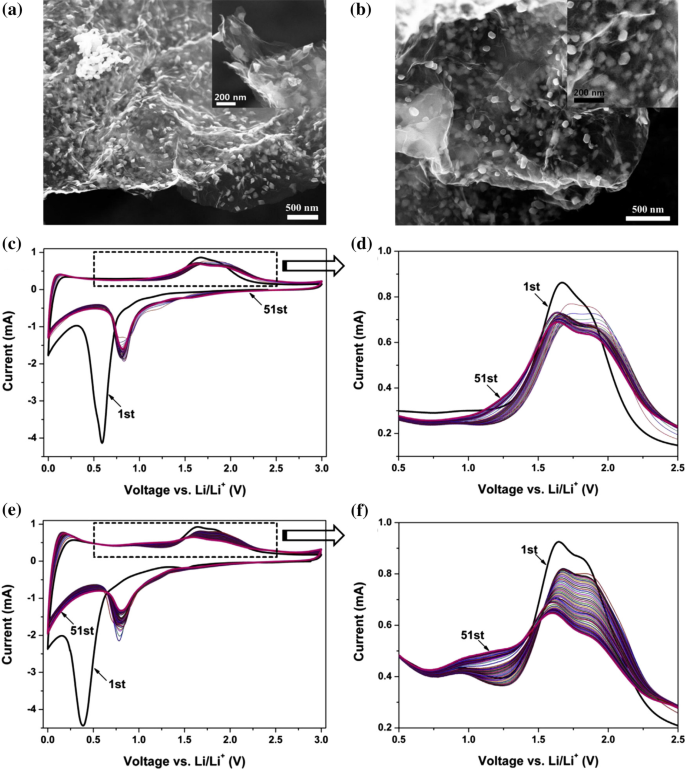
Nachdruck mit Genehmigung von [11]. Urheberrecht, Elsevier B.V.
REM-Bilder von a Fe2 O3 /Graphen und b K-FeO/Graphen und der Einschub in (a , b ) sind ihre vergrößerten Bilder. Wiederholte zyklische Voltammogramme von c , d das Fe2 O3 /Graphenelektrode und e , f die K-FeO/Graphen-Elektrode. Zwei Elektroden wurden für die 51. Zyklen kontinuierlich mit einer Potential-Sweep-Rate von 0,5 mV s −1 . gescannt .
In-situ-Röntgenbeugung
Durch in-situ-Röntgenbeugung (XRD) können die Informationen über die Echtzeit-Strukturänderung während des Reaktionsprozesses der Probe und eine große Menge vergleichbarer Informationen in kurzer Zeit erhalten werden. Es kann nicht nur die Strukturänderung der Probe während des Syntheseprozesses beobachten, es kann auch verwendet werden, um die entsprechende Strukturänderung der Probe bei verschiedenen Temperaturen unter Ladung/Entladung auf ein bestimmtes Potential zu erkennen, was sehr nützlich ist, um den tatsächlichen Reaktionsmechanismus zu verfolgen der Anode/Kathode in der Batterie. Darüber hinaus untersuchte unsere Gruppe die genaue Kenntnis der Gitterkonstanten von Wüstit, die für die Untersuchung seiner physikalischen und chemischen Eigenschaften bei hohen Temperaturen erforderlich ist. Das Fe1−x O wurde synthetisiert und durch ein Hochtemperatur-Röntgendiffraktometer (RINT2000-TTR, Rigaku Denki Co., Ltd.) mit parallelen Strahlen (Fig. 2a) zur Messung der spezifischen Beugungspeaks und der Beziehung zwischen der Zusammensetzung und der Gitterkonstante von . charakterisiert Wüstit bei hoher Temperatur wird ebenfalls untersucht. Der Syntheseprozess von Wüstit mit dem α-Fe (95 Gew.%) und Fe3 O4 (99 Gew.-%) Pulver als Ausgangsmaterial ist eine eutektoide Reaktion (Abb. 2b). Diese Reaktion kann zwischen 843,15 und 1673,15 K bei bestimmten Pco/Pco2 . ablaufen da der Wüstit unter 843,15 K instabil ist und die Reaktanten 19% Fe (Gew.%) und 81% Fe3 . sind O4 (Gew.%). Abbildung 2c zeigt das XRD-Muster der anfänglichen Reaktanten (Magnetit und Eisen). Die Versuchsbedingungen sind in Tabelle 1 beschrieben. Nach dem Spülen von Helium (He)-Gas (25 ml min −1 ) in den Ofen des XRD-Systems für 60 min, die Reaktanten wurden mit einer konstanten Geschwindigkeit von 10 °C min −1 . erhitzt bis die Temperatur der Proben 843,15 K erreichte, die sich auch in He-Atmosphäre mit einer Flussrate von 25 ml min −1 . befanden . Dann war das He-Gas erschöpft und CO/CO2 Gas mit einem bestimmten Verhältnis (z. B. 1:1 und 1:2) wurde in den Ofen gespült. Nach der Hochtemperaturkalibrierung durch Schmelzen von Au-Flakes konnte die tatsächliche Temperatur des Probenhalters ermittelt werden. Von 843,15 K auf die gewünschte Temperatur (1265,28 und 1365,28 K) betrug die Heizrate 2 °C min −1 und das XRD wurde verwendet, um die Probe zur Bestätigung der Wüstit-Phase zu messen. Wenn die Probe bei der gewünschten Temperatur aufbewahrt wurde und die meisten davon die Wüstit-Phase waren, wurden die Beugungswinkel der Wüstit-Kristallebenen über einen Zeitraum von 240–420 Minuten gemessen, dann die Temperatur der Probe um 50 °C erhöht und bei dieser Temperatur belassen für 60 min. Nach diesen Verfahren wurde die Temperatur der Probe auf die zuvor gewünschte Temperatur gesenkt und die XRD-Messung für Beugungswinkel der Kristallebenen von Wüstit wurde erneut in einem Zeitraum von 180–240 Minuten durchgeführt.
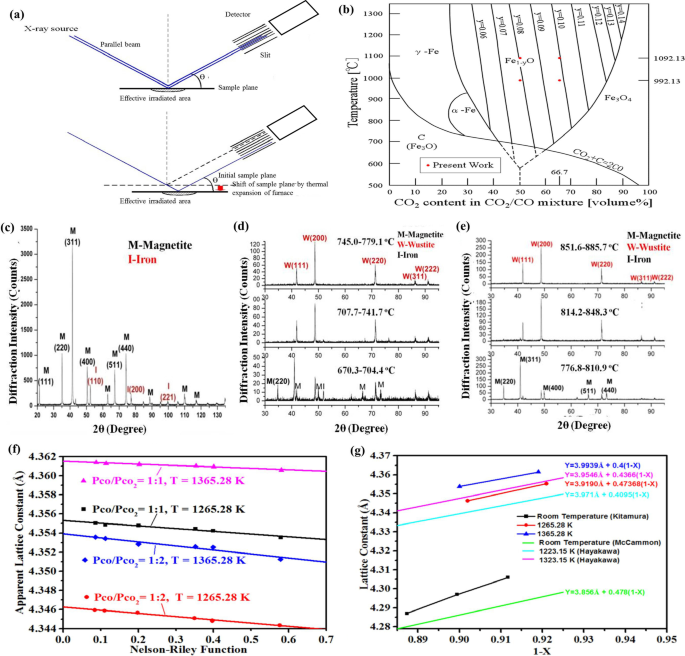
a Schematische Darstellung von In-situ-XRD. b Gleichgewicht zwischen Fe, Fe1−y O, Fe3 O4 , CO, CO2 , und Kohlenstoff. c XRD-Muster der anfänglichen Reaktanten. d XRD-Muster der bei Pco/Pco2 . synthetisierten Probe = 1:1. e XRD-Muster der bei Pco/Pco2 . synthetisierten Probe = 1:2. f Die verschiedenen NR-Funktions-scheinbaren Gitterkonstanten von Wüstit, synthetisiert bei verschiedenen Temperaturen und Pco/Pco2 . g Die Ergebnisse der Gitterkonstanten von Wüstit
Die Gitterkonstante kann durch eine lineare Extrapolation der scheinbaren Gitterkonstanten auf Null dieser Funktion erhalten werden, dh 2 Theta = 180°. Die Beugungspeaks der (111), (200), (220), (311) und (222) Kristallebenen von Wüstit sind in Fig. 2d, e indiziert, und das XRD-Muster der Probe wird bei Pco/Pco . erhalten 2 1:1 bzw. 1:2. Die Beziehung zwischen der scheinbaren Gitterkonstante und der Nelson-Riley-Funktion unter verschiedenen Pco/Pco2 und Temperaturen erhalten, wie in Fig. 2f gezeigt. Die geraden Linien stellen die Quadrate dar, die zu den Daten passen. Mit Hilfe dieser Geraden wurden die scheinbaren Gitterkonstanten auf den Nullpunkt der Nelson-Riley-Funktion extrapoliert. Daher, wie in Abb. 2f gezeigt:die Ergebnisse der wahren Gitterkonstanten, die bei verschiedenen Temperaturen und Pco/Pco2 . erhalten wurden sind 4,355 Å (1265,28 K, Pco/Pco2 = 1:1), 4.346 Å (1265.28 K, Pco/Pco2 = 1:2), 4.362 Å (1365.28 K, Pco/Pco2 = 1:1) und 4.354 Å (1365.28 K, Pco/Pco2 = 1:2). Wie in Abb. 2g gezeigt, steigt die Gitterkonstante mit der Zunahme von x von Fe1−x O, und je höher die Temperatur, desto größer die Gitterkonstante. Die Beziehung zwischen der Zusammensetzung und der Gitterkonstante von Wüstit bei hoher Temperatur kann wie folgt erhalten werden Gl. (3) und (4).
$${\text{a}}\,\left( { {\AA} } \right) =3,919 + 0,474\left( {1 - x} \right){ }\left( {1265,28\,{\text { K}}} \right)$$ (3) $${\text{a}}\,\left( { {\AA} } \right) =3,994 + 0,400\left( {1 - x} \right ){ }\left( {1365.28\,{\text{ K}}} \right)$$ (4)57 Fe-Mössbauer-Spektroskopie
Die 57 Die Fe-Mössbauer-Spektroskopie umfasst Eigenschaften des Kerns, einschließlich der Energieniveaustruktur des Kerns und der chemischen Umgebung, in der sich der Kern befindet. Daher kann es entsprechend angewendet werden, um die Wertigkeit von Atomen, die Ionizität chemischer Bindungen, die Koordinationszahl, die Kristallstruktur, die Elektronendichte und die magnetischen Eigenschaften der Probe zu untersuchen. Die 57 Die Fe-Mössbauer-Spektroskopie findet breite Anwendung in den Bereichen Chemie und Werkstoffe. Hier erläutern wir die 57 Fe-Mössbauer-Spektroskopie zur Charakterisierung von Eisenoxiden. Die 57 Die Fe-Mössbauer-Spektroskopie wird verwendet, um verschiedene Eisenoxidphasen zu unterscheiden und zu charakterisieren und die lokale Umgebung von Fe-Atomen im Kristallgitter zu überwachen [39, 40].
Die Hyperfeinparameter wie Isomerenverschiebung (IS), Quadrupolaufspaltung (QS), Quadrupolverschiebung (ɛ Q ) und hyperfeines Magnetfeld (B hf ) erhält man durch Analyse der Lage der Spektrallinien im Mößbauer-Spektrum [41, 42]. Aus der Breite und Asymmetrie der Spektrallinien kann auf die Eigenschaften der Probe geschlossen werden. Durch Temperatur- und Feldabhängigkeit der Hyperfeinparameter lassen sich auch wertvolle Parameter ableiten.
Aldonet al. [42] untersuchten die Lithium-induzierte Umwandlungsreaktion von Fe1−x O mit 57 Fe Mössbauer-Spektroskopie. Die Hyperfeinparameter (IS und QS) sind eher charakteristisch für FeII-Spezies in antiferromagnetischem (TN = 198 K [43]) Fe1−x O, zeigt eine typische paramagnetische Absorption bei Raumtemperatur (RT). Wie in der 57 angegeben Fe-Mössbauer-Spektrum (Abb. 3a), es gibt drei verbreiterte Dubletts mit einem IS ~ 1 mm s −1 und QS im Bereich von ~ 0,50 bis 1,50 mm s −1 , und ihre relativen Flächen werden aus dem magnetischen Beitrag von α-Fe korrigiert. Die Absorptionsintensitäten betragen 42, 26 und 15%. Ein viertes Dublett zentriert bei IS ~ 0.55 mm s −1 , QS ~ 0,90 mm s −1 mit einer relativen Fläche von 11 % ist für FeII I . üblich Spezies, wie in nichtstöchiometrischem Fe1−x . erwartet O. Vom FeII/FeII I Verhältnis wird die Zahl der offenen Stellen auf ~ 0,057 ± 0,008 geschätzt, die durch XRD-Charakterisierung auf 0,050 geschlossen wird. Schließlich befindet sich das Dublett bei QS ~ 1.68 mm s −1 und IS ~ 0 mm s −1 , entsprechend α-Fe, leistet einen Beitrag von ~ 6%.
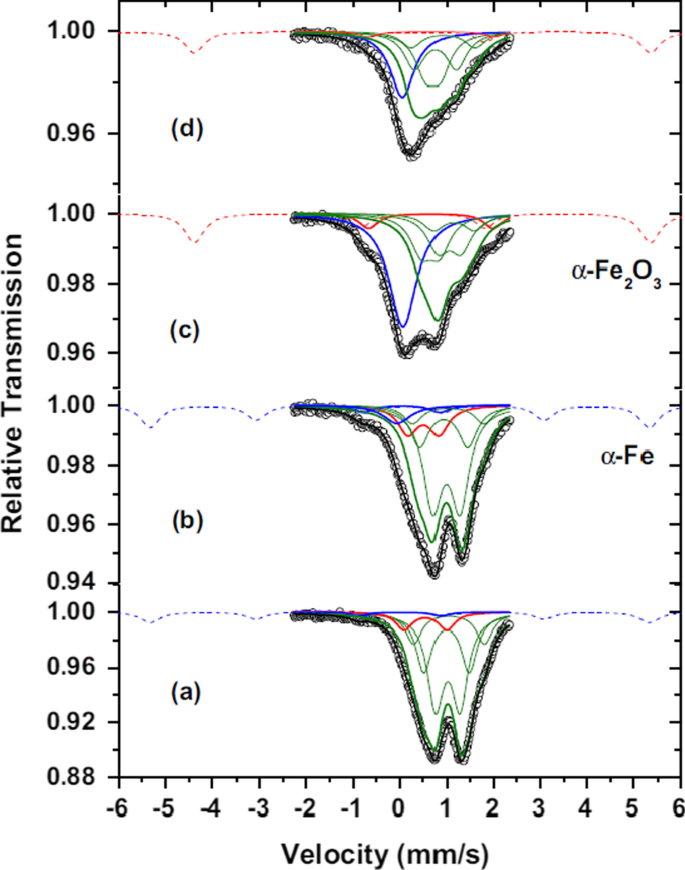
Nachdruck mit Genehmigung von [42]. Urheberrecht, Elsevier Masson SAS
Vergleich von 57 Fe-Mössbauer-Spektren bei 300 K des reinen Fe1−x O-Material (a ), nach einer Aufnahme von 1 Li (b ) und das Ende der Entladung bei 2,16 Li (c ). Grüne Beiträge werden FeII zugeschrieben. Die dickere grüne Linie ist die Summe der dünneren, die dem nicht umgesetzten Fe1−x . entspricht O. Die gestrichelte blaue Linie entspricht dem erwarteten steigenden Beitrag von α-Fe von (a ) bis (c ). Im Fall von (c ), magnetisches Sextett wurde als Orientierungshilfe für das Auge leicht verschoben. Spektrum (d ) entspricht dem Ende der ersten Ladung bei 0,94 Li ohne α-Fe-Beitrag, aber das blaue Singulett nanoskaliges metallisches ε-Fe 0 ist noch vorhanden.
Vergleich mit Fe2 O3 und Fe3 O4 , die spezifische Kapazität von Fe1−x O ist am niedrigsten. Außerdem ist sein Syntheseverfahren komplizierter. In den meisten Fällen ist die Reduktionsreaktion bei hoher Temperatur unvermeidlich [3,4,5,6, 9, 11]. Als Ergebnis ist Fe1−x O ist keine ideale LIBs-Anode im Vergleich zu Fe2 O3 oder Fe3 O4 .
Fe2 O3 Basierend auf Nanostrukturen
Unter diesen Eisenoxiden, insbesondere Fe2 O3 , zieht aufgrund der hohen theoretischen Kapazität, die 1000 mAh g −1 . erreichen kann, die Aufmerksamkeit vieler Forscher auf sich [44]. Zusätzlich Fe2 O3 hat entscheidende Vorteile, wie hohe Korrosionsbeständigkeit, niedrige Produktionskosten, Umweltfreundlichkeit, Nichtbrennbarkeit, Ungiftigkeit und hohe natürliche Verfügbarkeit [45]. Aufgrund dieser hervorragenden Eigenschaften ist Fe2 O3 ist vielversprechend für Anwendungen in der Anode von LIBs [46,47,48,49,50]. Hier ein kurzer Überblick über die jüngsten Entwicklungen zur Synthese, Charakterisierung und elektrochemischen Leistung von Fe2 O3 basierte Nanostrukturen bereitgestellt.
Synthese und Charakterisierung
In den letzten zehn Jahren wurden enorme Anstrengungen unternommen, um synthetische Methoden von Fe2 . zu erforschen O3 basierte Nanostrukturen. In diesem Abschnitt haben wir Synthesemethoden für Fe2 . ausgearbeitet und zusammengefasst O3 Nanostrukturen, einschließlich Gasphasenabscheidung [51], lösungsbasierte Verfahren [52], elektrochemische Verfahren [53], thermische Behandlung [54] und andere Verfahren [55, 56]. Außerdem haben wir verschiedene Synthesemethoden verglichen.
Außerdem sind die 57 Fe-Mössbauer-Spektroskopie-Charakterisierung von Fe2 O3 Nanostrukturen wird ausführlich beschrieben. Da nur bestimmte Kerne Resonanzabsorption haben, ist die 57 Die Fe-Mössbauer-Spektroskopie wird nicht durch andere Elemente gestört. Die Reichweite der 57 Die Fe-Mössbauer-Spektroskopie, die von einer extranuklearen Umgebung beeinflusst wird, liegt im Allgemeinen innerhalb von einigen Nanometern, so dass sie sehr gut zur Charakterisierung von Nanostrukturen geeignet ist.
Gasphasenabscheidung
Die Gasphasenabscheidung wird häufig bei der Synthese vieler dünner Filme und anderer Nanostrukturen wie Fe2 . angewendet O3 und andere auf Eisenoxiden basierende Nanostrukturen. Chemische Gasphasenabscheidung (CVD), Atomlagenabscheidung (ALD), physikalische Gasphasenabscheidung (PVD), elektrolytische Abscheidung und reaktives Sputtern sind typische Verfahren der Gasphasenabscheidung [57,58,59,60].
ALD ist ein einzigartiger Weg, um dünne Filme mit hoher Kristallinität zu synthetisieren, und ein billigerer Weg als die Flüssigphasenabscheidung. Beim ALD-Verfahren wird die chemische Reaktion jeder Schicht direkt mit der ersten Schicht begleitet. Auf diese Weise wird pro Reaktionszyklus nur eine Schicht abgeschieden. Lin et al. [51] nutzten ALD, um ein hochwertiges ultradünnes α-Fe2 . abzuscheiden O3 Film auf TiSi2 Nanonetze. Die selbstorganisierten nanoporösen 3D-Dünnschichten wurden von Yang et al. [61] durch CVD und integriert in ein heterogenes Fe2 O3 /Fe3 C-Graphen. Als Anode von LIBs können ihre Kapazität und Zyklizität durch Abscheidung dieses dünnen Films stark verbessert werden. Cesar et al. [62] abgeschiedene dünne Filme aus siliziumdotiertem Fe2 O3 dendritische Nanostrukturen durch Atmosphärendruck-CVD (APCVD), die Fe2 . produzierten O3 Photoanoden, die Wasser unter sichtbarem Licht mit beispielloser Effizienz oxidieren. Das dendritische α-Fe2 O3 Nanostrukturen zeigten eine makroskopische Oberfläche von 0,5 cm 2 [62]. Das vertikal ausgerichtete α-Fe2 O3 Nanorods-Array wird von Wu et al. [63]. Darüber hinaus haben Jia et al. [64] nutzten eine Hochfrequenz-Sputter-Abscheidung, um α-Fe2 . herzustellen O3 ultradünne Filme.
Obwohl die Gasphasenabscheidung in der Lage ist, qualitativ hochwertiges Fe2 . herzustellen, O3 basierten Nanostrukturen hat es auch Nachteile. APCVD und MOCVD haben beispielsweise eine hohe Toxizität und Entflammbarkeit im Prozess der Vorläufer.
Lösungsbasierte synthetische Methode
Die lösungsbasierte Synthesemethode ist weit verbreitet und lässt sich leicht herstellen, um Fe2 . herzustellen O3 und andere Eisenoxide. Fe2 O3 mit verschiedenen Morphologien, wie Nanoblumen [65], Nanosphären [66], Nanopartikel [67], Nanostäbchen [68], Nanotubes [14], Nanorings [69], Nanobelts [70], Nanoflakes [71], Nanowires [72] , Nanofasern [73] und Mikroboxen [54] wurden durch lösungsbasierte Verfahren, zB hydrothermale, solvothermale und Sol-Gel-Ansätze, synthetisiert. Diese Methoden sind sehr einfach und verfügbar. Zhonget al. [65] nutzten eine solvothermale Methode zur Herstellung von Fe2 O3 Nanoblumen (Abb. 4) über einen Ethylenglykol-vermittelten Selbstorganisationsprozess. Vayssieres et al. [52] berichteten über das Wachstum von porösem Fe2 O3 Nanostäbchen-Anordnung auf fluordotiertem Zinnoxid (FTO)-leitendem Glas durch einen hydrothermalen Prozess. Durch hydrothermales Wachstum von α-Fe2 O3 Vorläufer auf SnO2 Nanodrahtstämme, ein neuartiges sechszähliges verzweigtes α-Fe2 O3 /SnO2 Heterostruktur (Abb. 5) wurde synthetisiert [74]. Es gibt eine andere einfache und wirtschaftliche Technik, die Sol-Gel-Methode, um Fe2 . zu synthetisieren O3 Nanostrukturen. Wooet al. [68] nutzten eine Sol-Gel-Methode, um α-Fe2 . zu erhalten O3 Nanostäbchen durch Reaktion von ubiquitärem Fe 3+ in umgekehrten Mizellen. Die durch diesen Mechanismus erhaltenen Nanostäbe haben eine geringe Dimensionalität und eine große Oberfläche, die auf Magnetit und Wüstit erweitert werden kann.
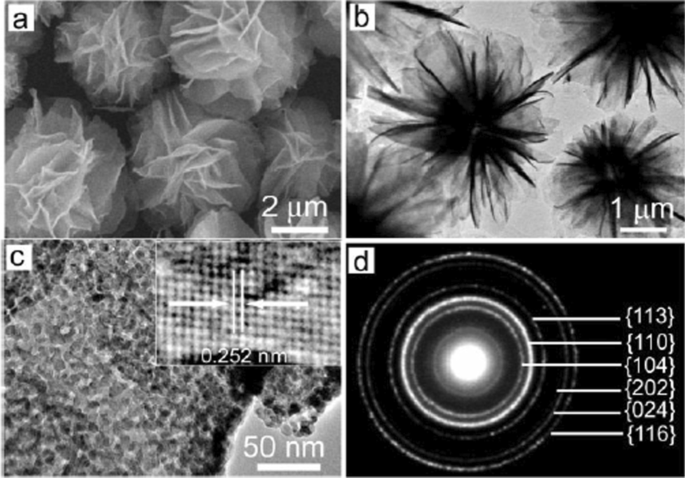
Nachdruck mit Genehmigung von [65]. Urheberrecht, Wiley-VCH
a SEM und b TEM-Bilder des erhaltenen α-Fe2 O3 . c Hochvergrößertes TEM-Bild des Blütenblatts der blütenartigen Struktur des so erhaltenen α-Fe2 O3 . d SAED-Muster des erhaltenen α-Fe2 O3 .
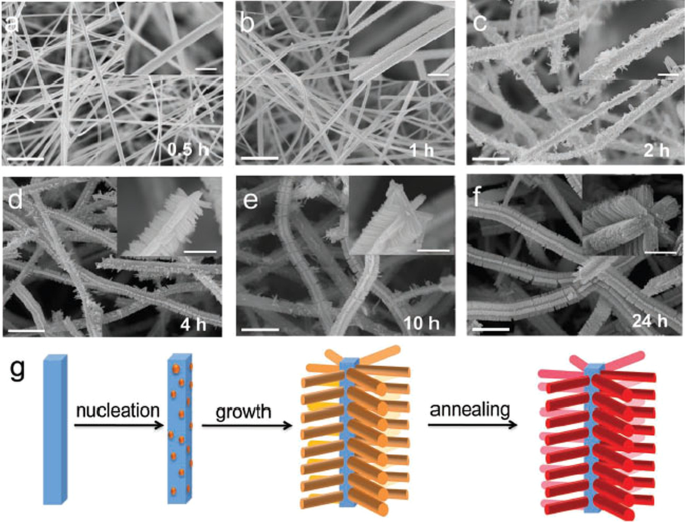
Nachdruck mit Genehmigung von [74]. Urheberrecht, Wiley-VCH
a –f REM-Aufnahmen der Produkte (nach dem Tempern) in verschiedenen Reaktionsstadien durch Einstellen der Reaktionszeit. Die Einschübe sind die entsprechenden vergrößerten REM-Bilder. Die Maßstabsbalken in den Abbildungen und Einfügungen betragen 2 µm bzw. 500 nm. g Schema der Bildung des hierarchisch aufgebauten α-Fe2 O3 /SnO2 Nanokomposit.
Elektrochemische Methode
Elektrochemische Methode wird verwendet, um Fe2 . zu synthetisieren O3 Nanostrukturen, z. B. die elektrochemische Abscheidung wird bei der Herstellung von Fe2 . angewendet O3 Nanopartikel [53]. Durch Anodisierung von Eisenfolie in Ethylenglykol-Elektrolytlösung mit entionisiertem (DI) Wasser und NH4 F bei einer Spannung von 30–60 V, α-Fe2 O3 Nanoröhren-Array wurde erhalten [75]. Darüber hinaus wird elektrochemische Anodisierung verwendet, um Fe2 . zu synthetisieren O3 Nanoröhren-Array [76]. Maoet al. [77] berichteten über die Synthese von Fe2 O3 Array mit elektrochemischer Abscheidung. In ihrer Forschungsarbeit wurde Eisen durch elektrochemische Abscheidung in AAO-Templatkanäle abgeschieden, dann wurde das AAO-Templat durch NaOH-Lösung entfernt und schließlich wurde das Array aus Eisen-Nanostäben in Fe2 . umgewandelt O3 Array. Das Merkmal dieser Forschung ist, dass durch Änderung der Abscheidungsdauer die Länge von Fe2 O3 Nanostäbe können fein gesteuert werden.
Thermische Behandlung
Die thermische Behandlung zur Synthese von Fe2 O3 beinhaltet zwei wesentliche Ansätze, thermische Oxidation und thermische Pyrolyse. Zhang et al. [54] hergestelltes Fe2 O3 Mikroboxen (Abb. 6) durch thermisch induzierte oxidative Zersetzung von Preußischblau (PB)-Mikrowürfeln bei 350–650 °C. Der Festkörperansatz wird im Vergleich zu der weit verbreiteten lösungsbasierten Methode einen einfacheren Weg für die großtechnische Synthese einheitlicher anisotroper Hohlstrukturen bieten. Fe2 O3 mit unterschiedlichen Morphologien wurden über kommandierende thermische Oxidationsparameter hergestellt. α-Fe2 O3 Nanostrukturen durch einfache thermische Behandlung von eisenbasierten Vorläufern werden vorgeschlagen. Rao und Zheng [71] verwendeten beispielsweise eine Wärmebehandlung, um dicht ausgerichtetes α-Fe2 . herzustellen O3 Nanoflocken-Array.
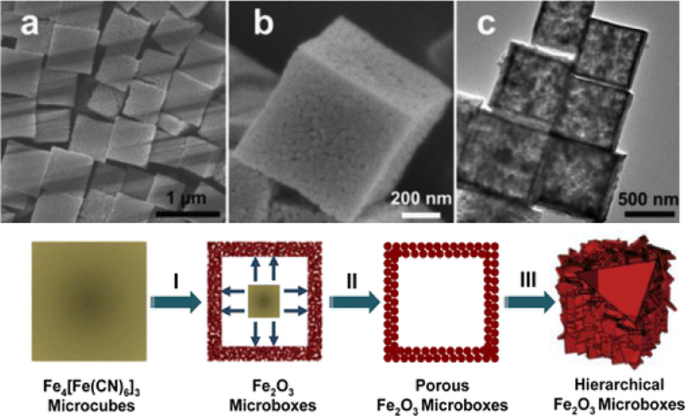
Nachdruck mit Genehmigung von [54]. Urheberrecht, American Chemical Society
a , b FESEM und c TEM-Bilder von hohlem Fe2 O3 Mikroboxen, erhalten bei 350 °C. d Schematische Darstellung der Bildung von hohlem Fe2 O3 Mikroboxen und die Entwicklung der Schalenstruktur mit steigender Kalzinierungstemperatur.
Thermische Pyrolyse ist eine weitere gängige Methode zur Abscheidung von Fe2 O3 dünne Filme. Duret et al. [78] wandten diese Methode an, um mesoskopisches α-Fe2 . zu erhalten O3 Blättchenfilme durch Ultraschall-Sprühpyrolyse, während die hergestellten Filme eine höhere Photoaktivität aufweisen als diejenigen, die durch herkömmliche Sprühpyrolyseverfahren hergestellt werden. Auch die Synthese von α-Fe2 O3 Nanoflocken, Nanoblumen, Nanodrähte und Nanostäbe werden durch Gasphasenabscheidung, Flüssigphasenabscheidung und thermische Behandlung beschrieben [63, 71, 72, 79].
Andere Methoden
Im Jahr 2016 haben Guivar et al. [55] zusammengesetzter leerer geordneter Maghemit (γ-Fe2 O3 ) Nanopartikel, die mit Nanohydroxyapatit (nanoHAp) funktionalisiert wurden, unter Verwendung einer typischen chemischen Kopräzipitationsroute. Bemerkenswert ist, dass das γ-Fe2 O3 funktionalisiert mit nanoHAp markiert als γ-Fe2 O3 @HAp wird ohne thermischen Prozess als Kalzinierung gebildet.
Es gibt viele Möglichkeiten, Fe2 . zu synthetisieren O3 , aber die meisten von ihnen sind nicht umweltfreundlich. 2019 haben Bashir et al. [56] entwickelte eine umweltfreundliche Methode zur Gewinnung von α-Fe2 O3 Nanopartikel, unter Verwendung von Persea Americana Samenextrakt. Sie verwendeten zwei verschiedene Vorstufen, um zwei Proben von α-Fe2 . herzustellen O3 , eine Probe (A), hergestellt aus Fe(NO3 )3 ·9H2 O, und eine andere Probe (B) präparierte FeCl3 ·9H2 O.
Die 57 Fe-Mössbauer-Spektren der Proben A und B, aufgenommen bei 300 K (Raumtemperatur (RT)) sind in Abb. 7 dargestellt. Die 57 Die Fe-Mössbauer-Spektroskopie ist eine sehr wertvolle Technik zur Untersuchung des lokalen magnetischen Verhaltens und des Oxidationszustands von Eisenatomen in einer bestimmten Matrix [80]. Beide Proben zeigten eine magnetische Ordnung und zeigten nur ein einzelnes Sextett, das einen magnetisch geordneten Zustand anzeigt. Tabelle 2 zeigt 57 Fe extracted Mössbauer parameters at RT, which lists IS, ɛ Q and B hf . Both samples’ B hf above 51 T are related to α-Fe2 O3 [81]. Furthermore, the values of ɛ Q is also consistent with α-Fe2 O3 . Both quadrupole interactions indicate Fe as Fe 3+ since the observed IS of 0.3653 mm/s and 0.3754 mm/s for the samples A and B, respectively, are typical for Fe 3+ [82]. Therefore, the negative values of quadrupole splitting indicate the weak ferromagnetic property of the samples A and B, the characteristic of pure α-Fe2 O3 phase.
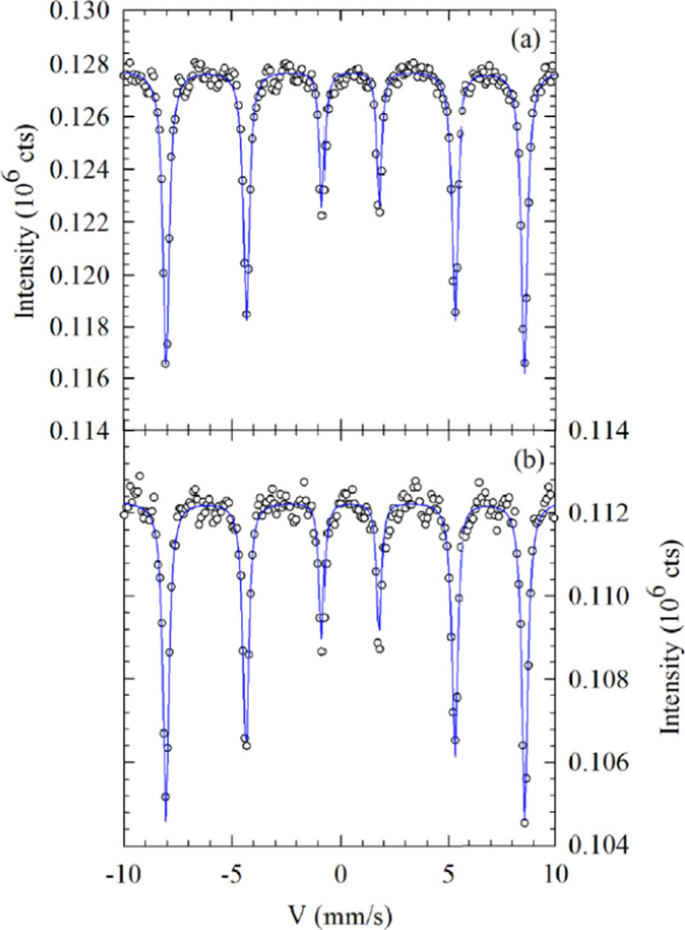
Reprinted with Permission from [56]. Copyright, American Chemical Society
57 Fe Mössbauer spectra recorded at room temperature for α-Fe2 O3 nanoparticles. a sample A (ferric nitrate as a precursor) and b sample B (ferric chloride as a precursor).
Electrochemical Performance
The charge/discharge cycling at the voltage window of 0.005–3.0 V (vs. Li + ) under a current density of 200 mA g −1 at RT is shown in Fig. 8a. During the initial discharge process, there is an obvious voltage platform of ~ 0.75 V, and it gradually moved to a voltage plateau of ~ 1.0 V, and remain stable in the second and fifth cycles. Meanwhile, an ambiguous plateau was observed at ~ 1.8 V in the charge process. The first discharge profile qualitatively resembles the results by Larcher et al. [83] and Morales et al. [84] on nanoparticle Fe2 O3 , and Wang et al. [14] on Fe2 O3 nanotubes. The cycling performance of three samples (hierarchical Fe2 O3 microboxes, Fe2 O3 microboxes and porous Fe2 O3 microboxes) is discribed in Fig. 8b. After 30 cycles, hierarchical Fe2 O3 microboxes exhibit the highest reversible capacity of 945 mA h g −1 , follow by 872 mA h g −1 for porous Fe2 O3 microboxes, and finally 802 mA h g −1 for Fe2 O3 microboxes. The results demonstrated that three samples display excellent cycling stability, and the morphology of nanostructured Fe2 O3 plays a significant role in determining the discharge characteristics.
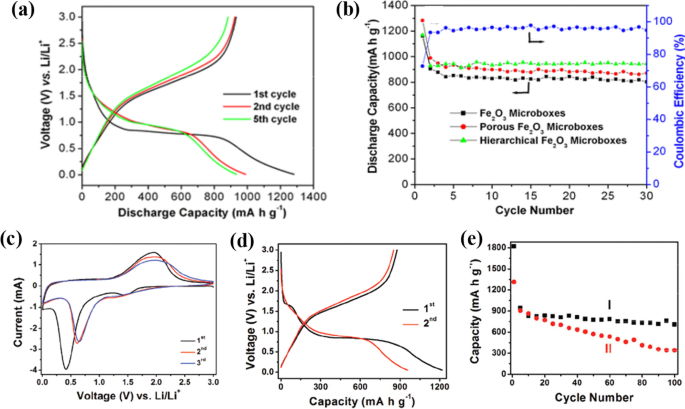
c –e Reprinted with Permission from [85]. Copyright, American Chemical Society
a Discharge − charge voltage profiles of porous Fe2 O3 microboxes obtained at 550 °C. b Cycling performance of Fe2 O3 microboxes (350 °C), porous Fe2 O3 microboxes (550 °C), and hierarchical Fe2 O3 microboxes (650 °C) and Coulombic efficiency of porous Fe2 O3 microboxes (550 °C) over the voltage range 0.01 − 3.0 V vs. Li/Li + at the same current density of 200 mA g -1 . (a , b Reprinted with Permission from [54]. Copyright, American Chemical Society). Electrochemical measurements of the sample. c CVs between 5 mV and 3 V at a scan rate of 0.5 mV s −1 . d Charge–discharge voltage profiles. e Comparative cycling performance of (I) the as-prepared α-Fe2 O3 hollow spheres and (II) α-Fe2 O3 microparticles. All the galvanostatic tests are performed at a constant current rate of 200 mA g −1 between 0.05 and 3 V.
Cyclic voltammogram (CV) is used to characterize the cells with α-Fe2 O3 nanoflakes anode in the 0.005–3.0 V under a slow scan rate (at RT). Li metal is used as the counter and reference electrodes [20], consistent with previously reported results [85]. CV curves of α-Fe2 O3 hollow spheres between 5 mV and 3 V at a scan rate of 5 mV s −1 are presented in Fig. 8c. There are apparent redox current peaks, and demonstrate good reversibility of electrochemical reaction. As shown in Fig. 8d, a distinct voltage plateau can be discovered at ~ 0.75 V, consistent with CV curves. The charge–discharge voltage profiles reflect the lithium storage capacity of α-Fe2 O3 . The first cyclic discharge capacity and the charge capacity is 1219 mA h g −1 and 877 mA h g −1 respectively, which lead to a relatively low irreversible capacity loss of 28%. In the second cycle, the Coulombic efficiency increased quickly to 89%. Cycling performance of two samples are demonstrated in Fig. 8e. The sample (I) exhibits excellent cyclic capacity retention from the second cycle onward. After 100 cycles of charge/discharge, the reversible capacity is still as high as 710 mA h g −1 . Compared with α-Fe2 O3 microparticles, the unique hierarchical α-Fe2 O3 hollow spheres apparently have enhanced Li storage performance, and a more stable cycling capacity retention and a higher reversible capacity are realized. This superior performance can be attributed to the thin nanosheet subunits that provide rapid and efficient transport of Li + , as well as the unique hollow interior that allows the material to effectively buffer the stress generated during charge/discharge process.
Iron oxides are cheap, abundant and environmentally compatible, and Fe2 O3 has excellent electrochemical performance. Some of the above studies have shown that Fe2 O3 based nanostructures can be an alternative anode to replace the presently used graphite in LIBs. The nanostructured Fe2 O3 has great potential in LIBs anode [86,87,88,89,90,91,92,93,94,95]. Recently, several studies about Fe2 O3 anode in asymmetric supercapacitors are reported [96,97,98,99,100]. However, low surface area and poor electrical conductivity are still two critical issues limiting the specific capacitance and power density of Fe2 O3 . For solutions of these problems, CNTs and CNFs are regarded as conductive matrices to load Fe2 O3 nanoparticles for realizing improved performance [101]. Table 3 summarizes some typical Fe2 O3 based nanostructures with their synthesis and electrochemical performance.
Fe3 O4 Based Nanostructures
Magnetite (Fe3 O4 ) and Fe3 O4 based nanostructure composites with pseudocapacitance, high theoretical capacity, environmental friendliness and low cost, are extensively applied to electromagnetic wave absorption [106], LIBs [3, 12, 15], biotechnological devices [107, 108], and supercapacitor [4, 109]. Fe3 O4 nanoparticles is one of the high-performance anodes in electrochemical devices. Unfortunately, Fe3 O4 nanostructures still face some problems, such as a severe volume variation (~ 200%) during the insertion and extraction of Li + and relatively low electrical conductivity, posing negative influence on the cycling stability [19, 110, 111]. From research results, we found that the structure and morphology of Fe3 O4 have a high influence on the electrochemical performance of Fe3 O4 and Fe3 O4 based composites.
Synthesis and Characterization
Recently, nanostructure engineering is demonstrated as a highly-effective approach to obtain improved electrochemical performance of Fe3 O4 and Fe3 O4 based composites. Therefore, various nanostructures including 0D nanoparticles [112], 1D nanorods/wires [113, 114], 2D nanoflakes/sheets [115, 116], 3D hierarchical/porous architectures [117, 118], and hybrid nanostructures of iron oxides [16] are proposed. The electrochemical performance of Fe3 O4 nanostructures can be optimized by rational design of their morphology, composition, porosity and surface characteristics.
Solution phase synthetic method is a facile and rapid way to obtain Fe3 O4 based nanostructures, because of the associative advantages, such as low synthesis temperature (always below 250 °C), easy control of morphology via adjusting hydrothermal conditions (e.g., PH, density of reactant and dosage of active agent, etc.). Solution phase synthetic method includes solvothermal synthesis [119], thermolysis [120], co-precipitation [121], sol–gel process [122, 123], micro-emulsion [124], etc. Simultaneously we compare the pros and cons of these methods.
Solvothermal Synthesis
Solvothermal synthesis, which reacts in a special closed reaction vessel, is one commonly used methods for synthesizing Fe3 O4 . In a hermetic environment, it is a facile method using aqueous solution as reaction medium at high temperature and high-pressure hermetic environment. Fe3 O4 nanostructures with various morphologies (0D, 1D, 2D and 3D) were synthesized applying this approach.
An et al. [119] obtained the Fe3 O4 /graphene nanowires by solvothermal synthesis and calcination with FeCl3 , NH4 VO3 and graphene as precursors. Phase transition of Fe3 O4 /VOx (FVO) after annealing was confirmed by XRD. For the XRD pattern of sample without annealing, all diffraction peaks of FVO and graphene decorated FVO correspond to FeVO4 ·1.1H2 O. For the XRD pattern of sample after annealing, there are no peaks of any vanadium oxides. And the inductive coupled high frequency plasma (ICP) result indicated that the molarity ratio of Fe and V is ~ 0.94:1, confirming the existence of amorphous vanadium oxide.
Mu et al. [125] reported dispersed Fe3 O4 nanosheets on carbon nanofiber by combing the electrospinning and solvothermal process. In this work, Fe3 O4 nanosheets are uniformly attached on the surface of carbon nanofiber with the diameter of about 500 nm.
Fe3 O4 nanoparticle with high specific surface area via FeCl3 and organic solvent ethanolamine (ETA) as precursors is reported by Wang et al. [126]. In this preparation, ETA is critical factor for compounding Fe3 O4 nanoparticles with high specific surface area, and Fe 3+ is gradually reduced to Fe 2+ by ETA during dissolution process, demonstrating that Fe 2+ increased as the increase of ultrasonication time. The ratio of ETA and FeCl3 has a large impact on the nanoscale grain size and specific surface area of Fe3 O4 . And the results showed that the grain size of 20–40 nm is achieved with 60 mL ETA and 6 mmol FeCl3 . When the amount of ETA is 80 mL, smaller nanoparticles (5–10 nm) are obtained.
Another representative work is reported by Chen et al. [127], in which graphene nanosheets decorated with Fe3 O4 nanoparticles (USIO/G) were synthesized using a facile solvothermal process. For the synthesis of USIO composite decorated with reduced graphene oxide (RGO), is used FeCl3 ·H2 O as precursor, then NaHCO3 and L-ascorbic acid were added to form USIO/G. In this process, L-ascorbic acid was oxidized to dehydroascorbic acid (DHAA) by some of Fe 3+ , which were reduced to Fe 2+ . Formation process of USIO/G is schematically shown in Fig. 9. The Fe3 O4 nanoparticles with uniform distribution, which are beneficial for electrical conductivity of graphene, mitigation of volume expansion of Fe3 O4 , and facilitating Fe3 O4 particles into the electrolyte.
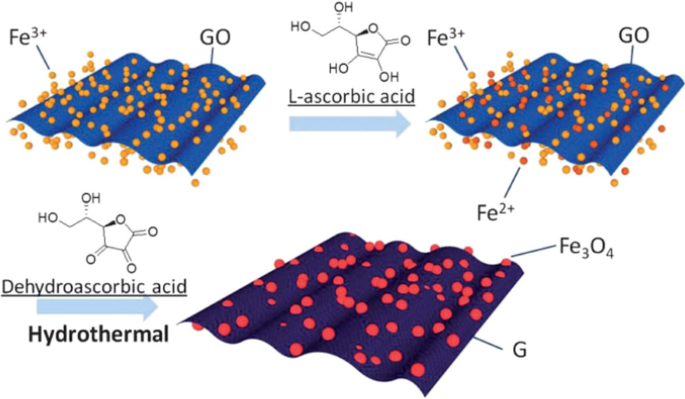
Reprinted with Permission from [127]. Copyright, Royal Society of Chemistry
Schematic illustration of the formation process of USIO/G.
Xionget al. [128] a kind of hierarchical hollow Fe3 O4 (H-Fe3 O4 ) microspheres prepared by controlled thermal decomposition of iron alkoxide precursor. In a classical reaction, ethylene glycol (EG) serves as reduction reagent that partly reduces Fe 3+ to Fe 2+ with sodium acetate (NaAc), and polyvinylpyrrolidone (PVP) [128]. For this synthesis, PVP served as a surface stabilizer, which has important role in the formation and transformation of hollow interiors.
With the development of solvothermal synthesis, it emerging as an efficient method with the advantages of low energy consumption, little reunion and easy to control shape, etc. Chen et al. [129] synthesized poly (acrylic acid) (PAA)-entangled Fe3 O4 nanospheres by a facile solvothermal method. In their synthesis, the ethylenediamine is crucial to the controlling of the uniformity of nanospheres, and the PAA molecules served as carbon source that transforms into the carbon matrix by heating treatment in inert atmosphere. As shown in SEM image of the prepared C-Fe3 O4 nanospheres, very uniform spherical particles with a diameter of 150–200 nm are synthesized. Observed from SEM images in Fig. 10a, the nanospheres contain small irregular particles, and have a relatively rough surface. In the control experiment without ethylenediamine (EDA), the synthesized particles are much less uniform with a wider size distribution of 100–500 nm, allowing the formation of nanospheres with smaller size.
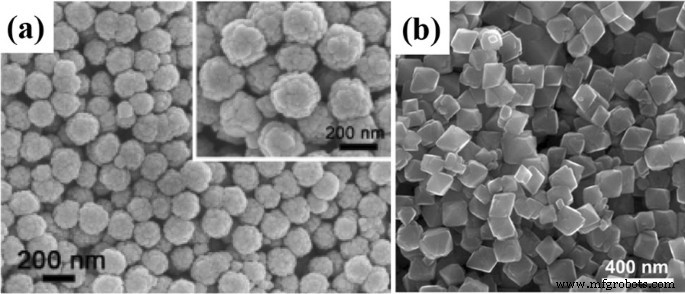
Reprinted with Permission from [130]. Copyright, Wiley–VCH
a SEM images of the Fe3 O4 nanospheres synthesized with ethylenediamine (EDA). The inset of (a ) shows a SEM image with higher magnification. (Reprinted with Permission from [129]. Copyright, American Chemical Society.). Physicochemical characterization of the octahedral Fe3 O4 nanoparticles. b SEM image showing the octahedral geometry of the iron oxide nanoparticles.
Co-precipitation
Due to its high cost-effectiveness, environmental friendliness, and facile synthesizing protocol, co-precipitation is a general approach for Fe3 O4 nanoparticles. Thus, in iron based rechargeable battery systems, Fe3 O4 nanomaterials are especially suitable for large-scale electrochemical applications to solve the energy requirement of the modern society.
Li et al. [121] proposed Fe3 O4 polyhedron as LIBs anode for alkaline secondary batteries by a co-precipitation. Annealing temperature makes a high effect on the physical and electrochemical performance of Fe3 O4 nanomaterials. The 700 °C-annealed Fe3 O4 exhibited a higher electrochemical performance, such as a higher specific discharge capacity of 604.2 mA h g −1 with a charging efficiency of 83.9% at 120 mA g −1 . Ooi et al. [130] demonstrated octahedral Fe3 O4 nanoparticles using a facile solvothermal route. Scanning electron microscope (SEM) image of Fe3 O4 nanoparticles is shown in Fig. 10b, which depicts that octahedral Fe3 O4 nanoparticles with an average length of 93 ± 18 nm were prepared by the hydrothermal method, showing a roughly Gaussian size distribution. Then, the crystal structure of octahedral nanoparticles can be further evaluated by HRTEM, and the composition of the bulk sample was further characterized by XRD and X-ray photo electron spectroscopy (XPS).
Thermolysis
The thermolysis is small monodisperse magnetic nanocrystals synthesized by organic metal compounds in high boiling point solvents containing stabilizing agent. Previous Organic metal bodies include metal acetylacetone compounds, metal cupferron, or metal Carbonyl compounds, and usually choose fatty acids, oleic acid, or hexadecyl amine as a surfactant. Zhang et al. [120] reported ultrafine Fe3 O4 nanocrystals uniformly encapsulated in two-dimensional (2D) carbon nanonetworks through thermolysis of Fe(C5 H7 O2 )3 precursor at 350 °C under vacuum, which named as 2D Fe3 O4 /C nanonetworks. This facile process using low-cost precursor proposed a green approach for preparing Fe3 O4 /carbon composite. Additionally, compared with the reported Fe3 O4 /carbon composites, the particle size of Fe3 O4 is controllable and a size of ∼ 3 nm can be obtained.
Benefitting from synergistic effects of carbon nanonetworks with excellent electrical conductivity and ultrafine Fe3 O4 particles with uniform distribution, high reversible capacity, excellent rate capability and superior cyclability at the voltage of 0.01–3.0 V (vs. Li/Li + ) are obtained. Nanoparticles with unique iron oxide (Fe3 O4 ) cores and zinc oxide (ZnO) shells were prepared by Jaramillo et al. [131]. Fe3 O4 nanoparticle synthesized through a thermolysis method using Fe(C5 H7 O2 )3 as organic metal body presoma, triethylene glycol as surface active agent, and core–shell Fe3 O4 @ZnO nanoparticles were successfully synthesized using straightforward methodologies. The structural and optical properties of the materials were characterized using a combination of X-ray diffraction, electron microscopy, and light spectroscopy. Importantly, the purity of the core and shell phases in the Fe3 O4 @ZnO nanoparticles was confirmed by both XRD and TEM, and the ZnO shell was shown to increase the transparency of the core–shell nanoparticles relative to the single-component Fe3 O4 nanoparticles. Zhang et al. [132] demonstrated a high crystalline Fe3 O4 -graphene composite by one-step reaction of thermolysis. And they demonstrated that the attachment of iron-organic complex with graphene oxide (GO) sheets can facilely result in magnetic graphene composites via a time-dependent calcination process.
Sol–Gel Process
The specific method is using the metal alkoxide, metal mineral compound or a mixture of the above two substances to hydrolysis and polymerization, uniform gel gradually, then condense into a transparent gel, however, after drying and heating, finally the oxide ultrafine powders was received. Tang et al. [122] prepared nanostructured magnetite thin film by sol–gel method using inexpensive iron (II) chloride precursor. Fe3 O4 nanoparticles were prepared at 300 °C, however, α-Fe2 O3 is generated when temperature increased to 350 °C, and this result restricts its applications. Xu et al. [123] proposed magnetite nanoparticles by virtue of sol–gel process combined with annealing in vacuum at 200–400 °C using nontoxic and low-cost ferric nitrate. In their study, Fe3 O4 nanoparticles with various sizes can be synthesized facilely through varying the annealing temperature.
Micro-emulsion Method
Micro-emulsion is composed of two mutual miscibility of liquid mixture of thermodynamic stability and isotropy dispersion, one of these or two kinds of liquid called micro area, and fixed by interface layer of the surfactant molecules. The key factors controlling the reaction solution contain concentration, pH value, reaction time and temperature. Micro-emulsion as a rapid expansion of new technology possesses many advantages. For example, high purity and uniform particle size distribution molecular dopant was synthesized at low temperature and simple reaction process. But there are also some shortcomings, for instance, the reaction system mostly contains organic solvents, which leads to high cost, pollution of environmental health and long reaction time. The prepared Fe3 O4 nanoparticles have excellent catalytic performance for the synthesis of quinoxaline in different solvents. Novel core–shell magnetic Fe3 O4 /silica nanocomposites with triblock-copolymer grafted on their surface (Fe3 O4 @SiO2 @MDN) were successfully synthesized by combining sol–gel process with seeded aqueous-phase radical copolymerization approach [133]. The Fe3 O4 @SiO2 @MDN microspheres were synthesized in following three steps. Firstly, the initial magnetic Fe3 O4 microspheres were synthesized by a solvothermal reaction. Then a sol–gel process was utilized to prepare silica coated Fe3 O4 microspheres (Fe3 O4 @SiO2 ), and a thin amorphous silica layer was formed on Fe3 O4 microspheres. Afterward, the Fe3 O4 @SiO2 microspheres were modified by 3-(methacryloxypropyl) trimethoxysilane (MPS). Finally, the triblock copolymer was fabricated by aqueous phase radical copolymerization reaction among MPS, divinylbenzene (DVB) and N-Vinyl-2-pyrrolidone (NVP) on the surface of Fe3 O4 @SiO2 . The magnetic Fe3 O4 particles with narrow size distribution have nearly spherical shape and smooth surface. Li et al. [124] reported hexagonal and triangular monodisperse Fe3 O4 nanosheets by a two-step microemulsion solvothermal approach, in which the uniform Fe3 O4 nanoparticles are prepared and then these hydrophobic nanocrystals are dispersed in a uniform microemulsion environment as “seeds” for further re-growth through a secondary solvothermal process. In the first step, near-spherical monodisperse 7–8 nm Fe3 O4 nanoparticles were formed through a kinetically controlled process. In the second step, the formation of anisotropic Fe3 O4 nanosheets is a thermodynamically controlled process and all the exposed surfaces of the triangular and hexagonal nanosheets are (111) crystal planes, which have the lowest surface energy for FCC Fe3 O4 .
Other Methods
Physical methods are also significant ways to prepared Fe3 O4 nanostructure for anode of LIBs. Several advantages, such as good crystallization, fine-tuned particle size, and high purity of products are highlighted in recent literatures. But these methods usually demand advanced and expensive equipment, result in a higher cost, poor dispensability of particles dispersion, and agglomeration of nanostructures. For instance, Du et al. [109] fabricated activated carbon (AC)-Fe3 O4 nanoparticles asymmetric supercapacitor, and Fe3 O4 nanostructure was prepared by microwave method. The precursor, FeSO4 ·7H2 O and NH3 ·H2 O mixed solution, was heated in microwave oven. The black precipitate was separated by magnet and washed repeatedly with DI water. The resulted microstructural properties of prepared nanoparticle were characterized by nitrogen adsorption (Quantachrome NOVA 2000), XRD and SEM [109]. Chen et al. [127] synthesized graphene nanosheets decorated with ultra-small Fe3 O4 nanoparticles (USIO/G). Seo et al. [134] reported an integrated usage of magnetic particles in microalgal downstream processes, specifically microalgal harvesting and lipid extraction through one-step aerosol spray pyrolysis and applied in microalgal harvesting and serial microalgal lipid entrapment. TEM/EDS, XPS, and FT-IR analysis suggested that the cationic and lipophilic functionalities arose from not fully decomposed PVP, due to the short residence time in the reactor. Kang et al. [135] proposed Fe3 O4 nanocrystals confined in mesocellular carbon foam (MSU-F–C) by a “host–guest” approach and applied it as LIBs anode. In this study, a precursor of Fe(NO3 )3 ·9H2 O is impregnated in MSU-F–C having uniform cellular pores with a diamter of ~ 30 nm, followed by heating treatment at 400 °C for 4 h in argon (Ar) atmosphere. Fe3 O4 nanocrystals with size of 13–27 nm were fabricated inside the pores of MSU-F–C. The existance of the carbon most likely allows the reduction of some Fe 3+ to Fe 2+ ions by a carbothermoreduction process. The physical performance and pore structure of MSU-F–C and Fe3 O4 -loaded composites were characterized with nitrogen sorption, and the composites have high capacities of ∼800–1000 mA h g −1 at 0.1 A g −1 (∼0.1 C rate), high rate capability and good cycling performance.
Application
Fe3 O4 possesses lots of unique properties, and is highly promising for applications in LIBs and supercapacitors [136,137,138,139,140,141,142]. Table 4 summarizes some applications.
Li-Ion Batteries
Due to conversion reaction of Fe3 O4 during charge/discharge process and other advantages, the Fe3 O4 is usually studied and applied as LIBs anode [143,144,145,146,147]. For TMOs, they have higher theoretical capacity (~ 500–1000 mA h g −1 ) than conventional graphite (about 372 mA h g −1 ). Furthermore, Fe3 O4 has superior conductivity compared with other transition metal oxides. Thus, it is well-focused by recent studies. It has been reported that composite electrodes with graphene have high performance due to their large surface area, high electrical conductivity and adaptive or flexible structure for high reliability. Qiu et al. [3] reported a kind of composite anode composed of ultra-dispersed Fe3 O4 nanoparticles (3–8 nm) and RGO sheet. It has excellent cyclic performance (624 mA h g −1 for up to 50 charge/discharge cycles at a current density of 0.1 A g −1 ), and good specific capability (624 and 415 mAh g −1 at 0.1 and 2.4 A g −1 , respectively) for LIBs. The obtained Fe3 O4 /RGO exhibited high and ultrastable Photo-Fenton activity (Fig. 11).
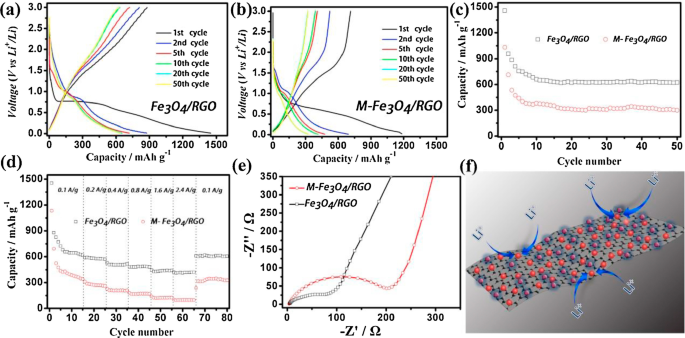
Reprinted with Permission from [3]. Copyright, Elsevier B.V
The charge/discharge curve of Fe3 O4 /RGO composites (a ) and mechanically mixed Fe3 O4 /RGO composites (M-Fe3 O4 /RGO) (b ) electrodes at constant current densities of 0.1 A g −1 . Cycling performance of Fe3 O4 /RGO composites and M-Fe3 O4 /RGO composites electrode at constant current densities of 0.1 A g −1 (c ). Rate capability of Fe3 O4/ RGO composites and physically mixed Fe3 O4 /RGO composites at the current densities between 0.1 A g −1 and 2.4 A g −1 (d ). Nyquist plots of the electrodes of Fe3 O4 /RGO sheet and mechanically mixed Fe3 O4 /RGO composites. All of the measurements were conducted using a voltage window of 0.01–3.0 V (e ). Schematic representation of the electrochemical reaction path on the Fe3 O4 /RGO composites (f ).
Pyrolyzed carbon is also a good “companion” for Fe3 O4 anodes. Apart from the facile protocol, porous structure formed by pyrolysis always exhibits high specific capacity of Fe3 O4 composite anode. Wang et al. [12] reported hollow N-doped Fe3 O4 /C nanocages with hierarchical porosities by carbonizing polydopamine-coated PB nanocrystals as LIBs anode (Fig. 12). The specific capacity of N-doped Fe3 O4 /C nanocages is ~ 878.7 mA h g −1 after 200 cycles at a current density of 200 mA g −1 , much higher than that of N-doped Fe3 O4 /C derived from pure PB (merely 547 mA h g −1 ). It is also desirable to design anisotropic structure of Fe3 O4 nanoparticles with carbon coated layer. Zhang et al. [19] reported a kind of carbon-coated Fe3 O4 nanospindles derived from α-Fe2 O3 nanospindles with length of about 500 nm and an axis ratio of ~ 4. Following by a hydrothermal synthesis method with glucose, the obtained LIBs anode delivered a high reversible capacity of ~ 745 mA h g −1 at C/5 and ~ 600 mA h g −1 at C/2.
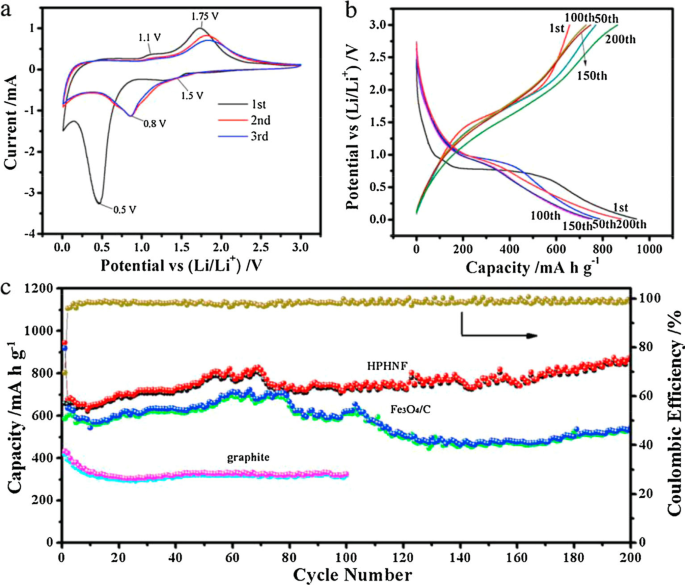
Reprinted with Permission from [12], Copyright, Elsevier Ltd
a CVs of the HPHNF during the first three cycles at 0.2 mV s −1 , b Galvanostatic charge/discharge profiles of the HPHNF electrodes for the 1st, 50th, 100th, 150th and 200th cycle at a specific current of 200 mA g −1 . c Cycling performance of the HPHNF nanocomposites, N-doped Fe3 O4 /C nanocomposites and graphite at a specific current of 200 mA g −1 . d Coulombic efficiency of HPHNF.
The most impressive work towards this field is probably the mesoporous iron oxide nanoparticle clusters with carbon coating reported by Lee et al. [148]. After a few cycles, the formation of SEI greatly enhanced the stability of interface between electrode and electrolyte. Electrochemical test exhibited a high specific capacity of 970 mA h g −1 for LIBs.
Supercapacitors
Fe3 O4 is a highly promising candidate for supercapacitor electrode because of its relatively high electrical conductivity, fast reversible redox reaction, low cost and eco-friendly nature [149,150,151,152]. Similar to batteries, high performance supercapacitors also require two factors:large specific surface area and long-term stability. Those two features usually were achieved by building some porous structures and carbon coated layers. Fe3 O4 nanoparticle with a high specific surface area was synthesized by Wang et al. [126] using a bottom up approach. Ferric chloride was firstly sonicated with ethanolamine and then processed through a solvothermal reaction. The obtained active nanomaterials showed a specific surface area of 165.05 m 2 g −1 and a specific capacitance of 207.7 F g −1 at 0.4 A g −1 .
Also, highly dispersed Fe3 O4 nanosheets on 1D CNFs is reported by Mu et al. [125]. The Fe3 O4 /CNFs composites showed a higher specific capacitance than pure Fe3 O4 in 1 M Na2 SO3 . To further enlarge the specific capacitance and cycle stability, hierarchically porous carbon spheres with Fe3 O4 using as supercapacitors exhibited high capacitivity of 1153 F g −1 at 2 A g −1 and high specific capacitance of 514 F g −1 at 100 A g −1 . In addition, the assembled asymmetric supercapacitor with double-shelled hollow carbon spheres and Fe3 O4 , has excellent cycling stability (96.7% retention after 8000 cycles) and high energy density (17–45 Wh kg −1 ) at a power density of 400–8000 W kg −1 [4].
Conclusion
Iron oxides (Fe1−x O, Fe2 O3 , Fe3 O4 ) based nanostructures have much higher specific capacities than those of commercial carbon based anodes. They are considered as highly promising candidates for LIBs anode. However, large irreversible capacity and low cycle stability are two serious problems that obstruct the application of iron oxides based nanostructures. In this review, we summarized the recent progress on novel iron oxides and their composites as LIBs anode and supercapacitor electrode. Several typical synthetic methods of various novel iron oxides based nanostructures are listed. By comparing the electrochemical performance of these various iron oxides based nanostructures, some strategies are expected to solve the problems of iron oxides based nanostructures.
Verfügbarkeit von Daten und Materialien
Not applicable.
Nanomaterialien
- Cervoz:Auswahl des richtigen Flash-Speichers für industrielle Anwendungen
- Optimierung der KI für eingebettete Anwendungen
- PPA für E-Mobilitätsanwendungen
- Drei große Fragen für jeden, der sich mit Energiespeichern beschäftigt
- Nanopartikel für die Krebstherapie:Aktuelle Fortschritte und Herausforderungen
- Zellbasierte Arzneimittelabgabe für Krebsanwendungen
- Fortschritte bei eisenoxidbasierten Nanostrukturen für Anwendungen in der Energiespeicherung
- Schätzung der Superkondensator-Energiespeicherung basierend auf fraktionierten Differentialgleichungen
- Bewertung von Graphen/WO3- und Graphen/CeO x -Strukturen als Elektroden für Superkondensatoranwendungen
- Fragen und Antworten:Öl- und Gasquellen zur Energiespeicherung