Platinbasierte Katalysatoren auf verschiedenen Kohlenstoffträgern und leitfähigen Polymeren für Direktmethanol-Brennstoffzellenanwendungen:eine Übersicht
Zusammenfassung
Nanopartikelmetalle auf Platin (Pt)-Basis haben große Aufmerksamkeit erregt und sind die beliebtesten Katalysatoren für Direkt-Methanol-Brennstoffzellen (DMFC). Die hohen Kosten von Pt-Katalysatoren, die langsame kinetische Oxidation und die Bildung von CO-Zwischenmolekülen während der Methanoloxidationsreaktion (MOR) sind jedoch große Herausforderungen im Zusammenhang mit Einmetall-Pt-Katalysatoren. Neuere Studien konzentrieren sich auf die Verwendung von Pt-Legierungen wie Fe-, Ni-, Co-, Rh-, Ru-, Co- und Sn-Metallen oder Kohlenstoffträgermaterialien, um die katalytische Leistung von Pt zu verbessern. In den letzten Jahren haben Pt- und Pt-Legierungskatalysatoren, die auf dem großen Potenzial von Kohlenstoffmaterialien wie MWCNT, CNF, CNT, CNC, CMS, CNT, CB und Graphen basieren, aufgrund ihrer signifikanten Eigenschaften, die zum hervorragenden MOR . beitragen können, bemerkenswertes Interesse gefunden und DMFC-Leistung. Dieses Übersichtspapier fasst die Entwicklung der oben genannten Legierungen und Trägermaterialien zusammen, um den Verbrauch von Pt zu reduzieren, die Stabilität zu verbessern und die elektrokatalytische Leistung von Pt in DMFC zu verbessern. Schließlich werden die einzelnen Katalysatoren und Träger in Bezug auf Morphologie, elektrokatalytische Aktivität, strukturelle Eigenschaften und Brennstoffzellenleistung erörtert.
Einführung
Die Brennstoffzellentechnologie hat weltweit große Aufmerksamkeit erregt. Brennstoffzellen (FCs) sind eine vielversprechende alternative Energieerzeugungstechnologie, die chemische Energie durch eine elektrochemische Reaktion in elektrische Energie umwandelt [1, 2]. Darüber hinaus liegt das Hauptaugenmerk der Brennstoffzellentechnologie für die Brennstoffzellentechnologie darauf, eine kostengünstige Produktion zu generieren, um so eine leistungsstarke Leistung des Brennstoffzellensystems zu erreichen und langlebige Materialien zu entdecken. Dennoch treten bei der aktuellen Brennstoffzellentechnologie häufig die Probleme auf, dass die Systeme hohe Eigenkosten und eine geringe Lebensdauer aufweisen [1]. Trotz ihres Versprechens als Brennstoffzelle haben Direkt-Methanol-Brennstoffzellen (DMFCs) Herausforderungen und Einschränkungen, was die Forscher dazu veranlasst, Methoden zur Verbesserung der DMFC-Effizienz und -Leistung zu untersuchen. Viele Probleme mit DMFCs wurden identifiziert und bleiben ungelöst, einschließlich des Übergangs von Methanolbrennstoff von der Anodenelektrode zur Kathodenelektrode [3,4,5] schlechte Leistung aufgrund der langsamen Kinetik, Instabilität des Katalysators und Wärme- und Wassermanagement [6,7,8].
In letzter Zeit gab es zahlreiche Untersuchungen zu Brennstoffzellen, einschließlich DMFC, Protonenaustauschmembran-Brennstoffzelle (PEMFC), Festoxid-Brennstoffzelle (SOFC) usw., die beliebte Brennstoffzellentechnologien sind. Als neuartige Energiequelle können DMFCs für mobile und stationäre Anwendungen eingesetzt werden [9, 10]. Auf dem Gebiet der Brennstoffzellen wurden viele Forschungsfortschritte erzielt. Unter den Brennstoffzellen wurden DMFCs in den letzten Jahren aufgrund ihrer vielen Vorteile, wie z Auswirkungen [17, 18]. Einige technische Herausforderungen für die Kommerzialisierung von DMFCs bleiben jedoch ungelöst, darunter Methanol-Crossover, niedrige chemische Reaktionsgeschwindigkeiten und Katalysatorvergiftung. DMFCs haben jedoch immer noch die Aufmerksamkeit vieler Forscher auf sich gezogen und sind aufgrund ihres Niedertemperaturbetriebs (DMFC-Systeme arbeiten bei 373 K) zu den beliebtesten Brennstoffzellen geworden. Aufgrund der Vorteile von DMFC, der hohen Energieeffizienz und des schnellen Startsystems, ist die DMFC-Technologie sehr gut geeignet, um als Energiequelle in Haushalten, Batterien in mobilen Geräten und als Fahrzeugkraftstoff eingesetzt zu werden [19,20,21,22]. Darüber hinaus könnte das Konzept der DMFCs weiter untersucht werden, um alternative Kraftstoffquellen wie Erdgas und Biomasse sowie die Vergärung von Agrarprodukten zu Ethanol zu finden, um die Abhängigkeit von unsicheren Energiequellen zu minimieren [14].
Bei der DMFC wird die Anodenseite mit Methanollösung versorgt, die einer Elektrooxidation zu Kohlendioxid (CO2 ) durch die Reaktion unten:
$$ {\mathrm{CH}}_3\mathrm{OH}+{\mathrm{H}}_2\to {\mathrm{CO}}_2+6{\mathrm{H}}^{+}+6{ \mathrm{e}}^{\hbox{-} } $$ (1)Während auf der Kathodenseite das Proton, der Sauerstoff (aus der Luft) zu Wasser reduziert wird:
$$ 3/2\ {\mathrm{O}}_2+6{\mathrm{H}}^{+}+6\ {\mathrm{e}}^{\hbox{-}}\to 3{\ mathrm{H}}_2\mathrm{O} $$ (2)Die Nettogleichung DMFC-Reaktion kann wie folgt zusammengefasst werden:
$$ {\mathrm{CH}}_3\mathrm{OH}+3/2{\mathrm{O}}_2\to {\mathrm{CO}}_2+2{\mathrm{H}}_2\mathrm{ O} $$ (3)In DMFC-Systemen gibt es zwei Arten von DMFC-Modi:aktive und passive Modi [23,24,25]. In einem aktiven DMFC-System wird der Auslassstrom des DMFC-Stacks durch die geschlossene Regelung des flüssigen Methanoleinsatzes rezirkuliert. Währenddessen wird das flüssige Methanol am Anodenstrom durch einen Methanolkonzentrationssensor gesteuert, der eine wichtige Rolle bei der Bereitstellung einer ausreichenden Einspritzung von zusätzlichem Methanol und Wasser spielt, um diesen Brennstoff basierend auf der Zielkonzentration wiederherzustellen. Es gibt verschiedene Arten von Methanolkonzentrationssensoren, die im DMFC-System verwendet werden, um die Methanolzufuhrkonzentration zu kontrollieren und aufrechtzuerhalten [17]. Üblicherweise wird das flüssige Methanol durch eine Schlauchpumpe auf die Anodenseite gefördert, während die sauerstoffhaltige Umgebungsluft durch ein Gebläse auf der Kathodenseite zugeführt wird [16]. In einem passiven Modus des DMFC-Systems wird das flüssige Methanol dem System kontinuierlich zugeführt. Dieses passive Konzept ist für DMFCs-Systeme sehr attraktiv [26,27,28]. Das Konzept von passiv bedeutet, dass das System völlig autonom ohne Unterstützungsgeräte arbeitet. Das Konzept der passiven DMFC bedeutet, dass das System völlig autonom arbeitet, ohne dass externe Geräte zum Pumpen von Methanol und Einblasen von Luft in den Stack benötigt werden. Im passiven Modus des DMFC-Systems wird die Katalysatorschicht durch Methanol und Sauerstoff als Reaktanten versorgt. Während der Methanoloxidationsreaktion (MOR) wird CO2 und Wasser wird auf passive Weise aus der Zelle entfernt, d. h. durch Diffusion, natürliche Konvektion, Kapillarwirkung usw. [20]. Die Passivmodus-DMFC scheint im Vergleich zur Aktivmodus-DMFC im Hinblick auf ein einfacheres, kompakteres Design und niedrige Kosten vorteilhafter zu sein. Das komplexe Systemdesign und die komplexe Steuerung können ein Nachteil der DMFC im aktiven Modus sein [21]. Aus dem Aspekt der praktischen Anwendung scheint die DMFC im aktiven Modus in Hochleistungssystemen geeigneter zu sein, während die DMFC im passiven Modus für die Verwendung in Niedrigleistungsanforderungen geeigneter ist [22].
Abbildung 1 zeigt den Aufbau und das Design einer Einzelzell-DMFC. Ein einzelliger DMFC-Stapel besteht aus einer fünfschichtigen Membran-Elektroden-Einheit (MEA), die von zwei Platten, die Anode und Kathode sind, eingeschlossen ist. Anodenseitig wird flüssiges Methanol (enthält Methanol und entionisiertes Wasser) und absolutes Methanol über eine Schlauchpumpe in den Kanal geleitet. Auf der Kathodenseite wird durch ein Rotameter Luft in die Brennstoffzelle gepumpt. Der Temperaturregler im DMFC-Stack wird verwendet, um die Arbeitstemperatur in der Zelle durch ein zusätzliches Heizgerät aufrechtzuerhalten. Das elektronische Lastgerät wird verwendet, um die Stromdichte auf verschiedene Niveaus zu ändern und die entsprechenden Werte der Spannung zu messen. Die Zellleistung wird von einer elektrochemischen Workstation überwacht, während die Produktion von CO2 als Endprodukt der Gesamtreaktion wird durch ein CO2 . gemessen Konzentrationsdetektor [23]. Bei einer Direkt-Methanol-Brennstoffzelle gibt es mehrere wichtige Betriebsparameter, die während der experimentellen Untersuchung berücksichtigt werden müssen, nämlich (i) Arbeitstemperatur, (ii) Methanolkonzentration und (iii) Eingangsdurchflussraten der zugeführten Methanollösung und Luft [23] . Abbildung 2a, b zeigt den aktiven bzw. passiven Modus der DMFC.
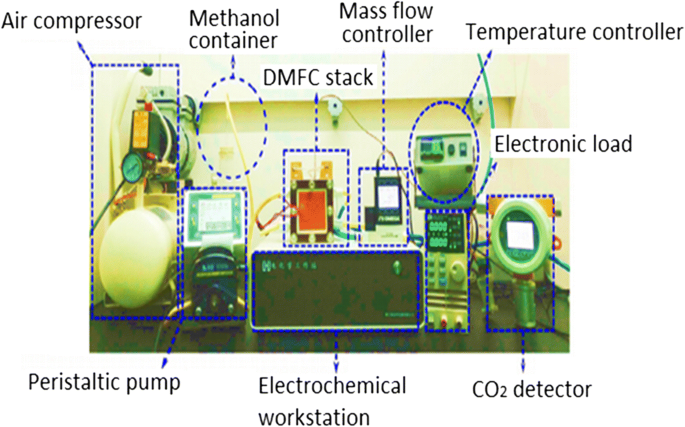
Ein allgemeiner Versuchsaufbau für Einzelzell-DMFC [23]
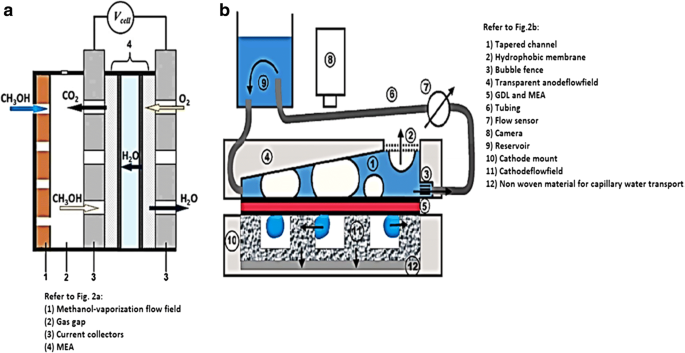
Schematische Darstellung von a aktiver Modus [24] und b passiver Modus [25] von DMFC
Dieser Aufsatz konzentriert sich auf die jüngsten Fortschritte in der Forschung und Entwicklung von Katalysatorträgern auf der Basis von Pt-Katalysatoren als Edelkatalysatoren in DMFC. Wir berücksichtigen die Aktivität von Pt-basierten Katalysatoren in Kombination mit Legierungen, Metallen, Übergangsmetallen, Metallcarbiden, Metallnitriden und verschiedenen kohlenstoffhaltigen Spezies, wie Graphen/Graphenoxid (G/GO), Kohlenstoffnanoröhrchen (CNT), Kohlenstoffnanofasern ( CNF), Carbon Nanocoil (CNC), Carbon Black (CB), Multiwall Carbon Nanotube (MWCNT) und Carbon mesoporous (CMS) Träger sowie leitfähige Polymere wie Polyanilin (PANi) und Polypyrrol (Ppy) als Träger Material. Viele Synthesemethoden können angewendet werden, um Pt-basierte Katalysatoren herzustellen. Die gebräuchlichsten Methoden, um nanoskalige Pt-Partikel zu erhalten, sind Imprägnierung [29,30,31,32,33,34], hydrothermale Techniken [35,36,37,38,39,40,41], Mikroemulsion [42,43, 44,45] und Reduktion [46, 47]. Im Allgemeinen kann das Herstellungsverfahren die Morphologie und Größe der Katalysatorteilchen beeinflussen; Daher ist die Auswahl der Methode zur Katalysatorsynthese sehr wichtig.
Leistung verschiedener Typen von Pt-basierten Katalysatoren
In den letzten zehn Jahren haben viele Forscher ihre Forschung auf die Entwicklung von Elektrokatalysatoren konzentriert, um seine elektrokatalytische Aktivität in Methanol-MOR für das DMFC-System zu verbessern [37, 38]. Platin (Pt) ist ein Einmetallkatalysator, der eine signifikant hohe katalytische Aktivität für den MOR zeigt. Reines Pt allein in einem DMFC-System kann jedoch leicht durch die Zwischenverbindung, dh Kohlenmonoxid (CO), vergiftet werden, und die hohen Kosten des Pt-Katalysators schränken seine kommerzielle Anwendung als Elektrokatalysator ein, wodurch die kinetische Geschwindigkeit der Methanoloxidation verringert wird im DMFC-System [48,49,50]. Diese drei Punkte sind die Haupthindernisse und Beschränkungen der alleinigen Verwendung von Pt als Elektrokatalysator für DMFC. Um diese Hindernisse zu überwinden, wurden jedoch mehrere Studien durchgeführt, um Elektrokatalysatoren auf Basis von Pt-Legierungen zu synthetisieren, um eine bessere elektrokatalytische Leistung mit weniger Pt-Verbrauch zu erzielen [11, 47, 51, 52]. Normalerweise können die durchschnittliche Größe von Pt-Partikeln und ihre Morphologie durch Rasteremissionsmikroskopie (REM) oder Transmissionselektronenmikroskopie (TEM) bestimmt werden, die die gängigsten Methoden im Bereich der Katalyse sind, die verwendet werden können, um die physikalischen Eigenschaften von Elektrokatalysatoren zu charakterisieren. Tabelle 1 zeigt die durchschnittlichen Partikelgrößen der Pt-Partikel mit verschiedenen Synthesemethoden, Eigenschaften und deren Leistungen.
Bimetallisches PtRu gilt aufgrund seines bifunktionellen Mechanismus und der Ligandeneffekte als der aktivste Katalysator [48, 53]. PtRu wird zu einer interessanten Katalysatorlegierung und wird bis heute mit vielen Kohlenstoffträgern verwendet. Die toxikologische Wirkung der Zugabe von Ruthenium (Ru)-Metall bleibt jedoch ungewiss [49]. Daher wurden Untersuchungen zu kostengünstigeren Legierungen durchgeführt, die Pt mit anderen unedlen Metallen mischen [49,50,51,52, 54,55,56,57], wie im Abschnitt „Leistung von Pt-basierten Legierungen“ beschrieben.
Leistung von Pt-basierten Legierungen
Aricoet al. [35] fanden heraus, dass zahlreiche Studien durchgeführt wurden, um die katalytische Aktivität von Pt-Katalysatoren im MOR zu erhöhen. In vielen Studien wurde das optimale Pt-Ru-Verhältnis als 1:1 identifiziert, und Partikelgrößen im Nanobereich sind die ideale Größe, um die Katalysatorausnutzung zu verbessern. Shi et al. [38] identifizierten in ihren Experimenten, dass 3:2 das optimale Verhältnis für Pt-Ru ist, um die katalytische Aktivität von MOR zu erhöhen. Abgesehen davon kann die elektrokatalytische Aktivität für die Methanol-Elektrooxidationsaktivität auch erhöht werden, wenn die PtRu-Elektrokatalysatorpartikel nanoskalige Partikel im Bereich von 2–4 nm sind. Paulaset al. [39] stimmte dieser Aussage zu. Wie wir wissen, zeigt Pt eine hohe Reaktivität gegenüber Methanolbrennstoff, was Pt-Metall zu einem idealen Elektrokatalysator für die Anodenelektrode in DMFC-Systemen macht. Trotzdem bildet sich während der MOR des Pt-Katalysators Kohlenmonoxid (CO), d. h. die Zwischenspezies, auf der Oberfläche der Pt-Partikel, die somit die Katalysatoroberfläche vergiftet [58,59,60,61]. Daher sind einige Anstrengungen erforderlich, um das Problem der Bildung giftiger Spezies auf der Oberfläche der Pt-Partikel zu überwinden, damit sie die Bereiche des aktiven Zentrums von Pt nicht verdecken. Im Allgemeinen sind binäre Legierungen wie PtRu [62,63,64,65,66], PtRh [67,68,69,70,71], PtAu [72,73,74], PtSn [62, 63, 75, 76,77, PtNi [64,65,66,67,78,69], PtCo [70, 71, 78,79,80] und PtFe [81.82,83,84,85], werden häufig eingesetzt als Elektrokatalysatorkombinationen für die Anodenelektrode im DMFC-System. Es wird angenommen, dass die Zusätze dieser Metalle, wie Ruthenium (Ru), Zinn (Sn) und Rhodium (Rh), eine höhere katalytische Aktivität erzeugen.
Der Einbau von Nickel (Ni) in Katalysatoren auf Platinbasis bietet eine überlegene Leistung für MOR und DMFC. In der neuesten Forschung erklären Guerrero-Ortega und Mitarbeiter, dass die Zugabe von Ni in den Pt-Vulcan-Träger eine bedeutende Zunahme des Faradischen Stroms während der MOR um eine Größenordnung fördert, obwohl der Einsatz von Pt im bimetallischen Katalysator geringer ist [ 55] . Ihre experimentellen Ergebnisse legten auch nahe, dass die Zugabe von Ni einige strukturelle und elektronische Modifikationen fördert, die eine bessere Reaktionsleistung an der Elektrodengrenzfläche verbessern. In einer anderen Arbeit verbesserte der Einbau von Au in eine Pt-Legierung die elektrokatalytischen Aktivitäten aufgrund der sich ändernden elektronischen Struktur und der Verbesserung der elektrochemisch aktiven Fläche (ECSA) [47]. Während die Zugabe von Zinn (Sn) zu einer Pt-basierten Legierung eine Zunahme der elektrokatalytischen Aktivität zeigte, die stark durch den Einbau von Sn in sein Legierungssystem und oxidierte Formen beeinflusst wird, beschleunigte die Reaktion aufgrund des niedrigeren Oxidationspotentials leichter [ 56]. Auch die Zugabe von Cobalt (Co) zu einer Pt-basierten Legierung verbesserte die katalytischen Eigenschaften des PtCo (1:9)/rGO-Katalysators erheblich, der zehnmal höher als Pt/rGO war [51]. Die Zunahme der Stromdichte wird der höheren Dispersion von PtCo-Nanopartikeln auf der hydrophilen Natur des rGO-Trägers zugeschrieben, die die Wasseraktivierung fördert und zur Oxidation der COAds . führt auf Pt-Sites. Darüber hinaus fördert es gemäß dem bifunktionellen Mechanismus von Co die H2 O-Aktivierung erzeugt mehr -OH-Ionen und andere O2 -haltige Spezies zur Oxidation von CO-Zwischenprodukten Spezies auf der Pt-Stelle [57]. Dieser bifunktionelle Mechanismus von Co könnte auch für andere katalytische Übergangsmetalle in Richtung MOR genutzt werden. Der katalytische Oxidationsmechanismus von CO-Spezies zu CO2 in Gegenwart von PtCo-Katalysatoren lässt sich wie folgt zusammenfassen:
$$ \mathrm{Pt}+{\mathrm{CH}}_3\mathrm{OH}\to \mathrm{Pt}\hbox{-} {\mathrm{CO}}_{\mathrm{ads}}+4 {\mathrm{H}}^{+}+4{\mathrm{e}}^{\hbox{-} } $$ (4) $$ \mathrm{Co}+{\mathrm{H}}_2\ mathrm{O}\to\mathrm{Co}{\left(\mathrm{OH}\right)}_{\mathrm{ads}}+{\mathrm{H}}^{+}+{\mathrm{e }}^{\hbox{-} } $$ (5) $$ {\mathrm{PtCO}}_{\mathrm{ads}}+\mathrm{Co}{\left(\mathrm{OH}\right) }_{\mathrm{ads}}/{\mathrm{CO}}_2+\mathrm{Pt}+\mathrm{Co}+{\mathrm{H}}^{+}+{\mathrm{e}}^ {\hbox{-} } $$ (6)Darüber hinaus haben Löffler et al. [86] synthetisierten erfolgreich PtRu als Anodenkatalysator für DMFCs, wodurch der aktivste Elektrokatalysator für die Methanolelektrooxidation mit etwa 50 Atom-% Ru hergestellt wurde. Inzwischen haben Dinh et al. berichteten, [87] dass PtRu mit einem PtRu-Verhältnis von 1:1 ein stärkeres metallisches Verhalten und eine höhere elektrokatalytische Aktivität für die Methanoloxidation (MOR) aufweist. Die Leistung hängt mit diesen beiden Hauptfaktoren zusammen:(i) maximierte Katalysatoroberfläche und (ii) Katalysatoroberfläche mit maximaler Anzahl von Metalllegierungsstellen mit einem Atomverhältnis nahe 1:1. Beide dieser Gruppe zeigten auch hoch. Basierend auf dem bifunktionellen Mechanismus haben Aricò et al. [58] und Goodenough et al. [62] schlugen vor, dass die an den Pt-oberflächenaktiven Zentren gebildete CO-Zwischenverbindung zu Kohlendioxid oxidiert werden kann (CO2 ) durch aktive Sauerstoffatome, die auf den Sekundärelementen, beispielsweise Ru, Sn und Mo, im unteren Potentialbereich gebildet werden. Tabelle 1 fasst die Leistung verschiedener Typen von Pt-Legierungskatalysatoren zusammen, die von Forschern für MOR durchgeführt wurden. Nach dem bifunktionellen Mechanismus [88,89,90] kann die MOR auf geträgerten PtRu-Legierungskatalysatoren als die folgende Gleichung zusammengefasst werden. Pt ist ein aktiverer Katalysator für die Methanoladsorption als Ru. Daher folgt die Gesamtreaktion auf PtRu-Elektrokatalysatoren für die Methanoloxidationsreaktion dem bifunktionellen Mechanismus.
$$ \mathrm{Pt}+{\mathrm{CH}}_3\mathrm{OH}\to \mathrm{Pt}\hbox{-} {\mathrm{CH}}_3\mathrm{OH}\mathrm{ads }\to \mathrm{Pt}\hbox{-} {\mathrm{CO}\mathrm{H}}_{\mathrm{ads}}\to 3\mathrm{H}+3\mathrm{e}\hbox {-} \to \mathrm{Pt}\hbox{-} {\mathrm{CO}}_{\mathrm{ads}}+{\mathrm{H}}^{+}+{\mathrm{e}} ^{\hbox{-} } $$ (7) $$ \mathrm{Ru}+{\mathrm{H}}_2\mathrm{O}\to \mathrm{Ru}\hbox{-} {\mathrm{ OH}}_{\mathrm{ads}}+{\mathrm{H}}^{+}+{\mathrm{e}}^{\hbox{-} } $$ (8) $$ \mathrm{Pt }\hbox{-} {\mathrm{CO}\mathrm{H}}_{\mathrm{ads}}+\mathrm{Ru}\hbox{-} {\mathrm{OH}}_{\mathrm{ads }}\to\mathrm{Pt}+\mathrm{Ru}+{\mathrm{CO}}_{2+}2{\mathrm{H}}^{+}+2{\mathrm{e}}^ {\hbox{-} } $$ (9) $$ \mathrm{Pt}\hbox{-} {\mathrm{CO}}_{\mathrm{ads}}+\mathrm{Ru}\hbox{-} {\mathrm{OH}}_{\mathrm{ads}}\to\mathrm{Pt}+\mathrm{Ru}+{\mathrm{CO}}_2+{\mathrm{H}}^{+}+{ \mathrm{e}}^{\hbox{-} } $$ (10)Bei diesem bifunktionellen Mechanismus wird Methanol zunächst dissoziiert und an Pt adsorbiert, anschließend zu COads . zersetzt und/oder formylähnliche Spezies -CHOAnzeigen durch Dehydrierungsreaktion (7). Gleichzeitig dissoziiert Wasser zu OHWerbung und an Ru-Stellen (8) adsorbiert. Dann adsorbieren die Spezies an Pt- und Ru-Zentren und verbinden sich zu CO2 Molekül (9) und (10). Die Reaktion zwischen Pt-COAds und Ru–OHads führt zu CO2 Evolution, wodurch aufgefrischte Pt- und Ru-Zentren erzeugt werden (Reaktion 10). Eine andere Arbeit von Ewelina Urbanczyk et al. [48] führten die Methanoloxidationsreaktion für PtNi-Katalysatoren in alkalischem Medium (1.0 M KOH) durch. Theoretisch ist die Methanoloxidationsreaktion im alkalischen Medium:
$$ {\mathrm{CH}}_3\mathrm{OH}+6\mathrm{OH}\to {\mathrm{CO}}_2+5{\mathrm{H}}_2\mathrm{O}+6{ \mathrm{e}}^{\hbox{-} } $$Die Reaktion startet an der Pt-Elektrode der DMFC zu Kohlendioxid. Während dieses Prozesses können sich Zwischenmoleküle (CO) bilden, die zu einer Vergiftung und Deaktivierung der aktiven Pt-Seite führen können. Dieses CO-Molekül ist ein Produkt der unvollständigen Oxidation von Methanol. Die unvollständige Methanoloxidation bildet das CO als Zwischenprodukt (Gl. 11). Die Elektrokatalysatoroberfläche kann auch Hydroxylgruppen adsorbieren (Gl. 6). Schließlich entsteht durch Desorption des Hauptprodukts Kohlendioxid (13). Das zweite Gift, das bei der Methanoloxidation entstehen kann, ist Methan. In diesem Fall kann die folgende Reaktion ablaufen (8). Die totale Oxidation der Zwischenform von Kohlenstoff zu Kohlendioxid in der elektrochemischen Reaktion ist wie folgt:
$$ 3\mathrm{Pt}+{\mathrm{CH}}_3\mathrm{OH}\to \mathrm{Pt}-\mathrm{COads}+4{H}^{+}+2\mathrm{Pt }+4e-+{H}^2O $$ (11) $$ \mathrm{Ni}+{H}_2O\to \mathrm{Ni}-\mathrm{OHads}+{H}^{+}+e - $$ (12) $$ \mathrm{Pt}-{\mathrm{CO}}_{\mathrm{ads}}+\mathrm{Ni}-\mathrm{OHads}\to {\mathrm{CO}} _2+{H}^{+}+\mathrm{Pt}+\mathrm{Ni}+e- $$ (13) $$ \mathrm{Pt}-{\mathrm{CH}}_3+\mathrm{Pt}- H\to 2\ \mathrm{Pt}+{\mathrm{CH}}_4 $$ (14) $$ \mathrm{Pt}-{\mathrm{CH}}_3+\mathrm{Ni}{\left(\ mathrm{OH}\right)}_2\to\mathrm{Pt}+{\mathrm{CO}}_2+\mathrm{Ni}+5{H}^{+}+5e- $$ (15)Derzeit untersuchen Forscher noch Legierungstechniken zur Verbesserung der katalytischen Aktivität von Pt-basierten Elektrokatalysatoren durch die Herstellung von ternären und quaternären Pt-Legierungen wie PtRuSn [91, 92], PtRuNi [93,94,95], PtRuMo [70, 96 , 97], quaternäres PtRuOsIr [79, 80] und PtRuIrSn [97, 98] aufgrund ihres ausgezeichneten Verhaltens in MOR und Entfernung der intermediären Spezies (CO), die sich auf der Pt-Oberfläche bilden. Die Zugabe des dritten und vierten Metalls in diesen ternären und quaternären Katalysatoren ist jedoch noch unbekannt. Darüber hinaus gibt es einige Einschränkungen und Herausforderungen bei der Herstellung der ternären und quaternären Legierungen. Die Optimierung der Katalysatormorphologie und der Katalysatorzusammensetzungen wird aufgrund vieler möglicher Kombinationen von Metallen und Zusammensetzungen schwierig zu erreichen. Viele Studien haben jedoch bewiesen, dass die Zugabe von drittem und viertem Metall die katalytische Aktivität bemerkenswert verbessert, die Stabilität des Katalysators erhöht und eine gute CO-Toleranz gegenüber Methanol-Elektrooxidation und DMFC-Anwendungen bietet.
Tsiouvaras et al. [99] führten elektrochemische Messungen an PtRuMo/C-Katalysatoren durch und fanden heraus, dass, obwohl alle ternären Katalysatoren gegenüber der CO- und Methanoloxidation aktiver waren als der binäre Katalysator, der mit H2 . behandelte Katalysator zeigten eine um ungefähr 15% verbesserte Leistung in Bezug auf die ternären Katalysatoren, die in He oder ohne Behandlung behandelt wurden. Im Jahr 2012 haben Hu et al. [100] synthetisierten erfolgreich einen überlegenen bimetallischen (PtNi) Elektrokatalysator, nämlich hohle mesoporöse PtNi-Nanosphären (HMPNNs). Aufgrund der einzigartigen Struktur der HMPNNs und ihrer großen elektrochemischen Oberfläche zeigte der Katalysator eine hervorragende katalytische Leistung im MOR mit einer deutlich verbesserten Pt-Nutzungseffizienz. Um das Jahr 2016 herum wurde die Arbeit von Yang et al. [101] untersuchten auch die Reaktivität synthetisierter bimetallischer PtFe-Elektrokatalysatoren, wobei sie beobachteten, dass die starke Wechselwirkung zwischen Pt und Eisen(Fe)-Metallen die Adsorptionsenergien von bimetallischen NPs verringern kann. Sie fanden auch heraus, dass die bimetallischen PtFe-Nanopartikel es vorziehen, durch die Fe-Atome an das Einzelleerstellen-Graphen adsorbiert zu werden, wenn sich sowohl Pt- als auch Fe-Atome auf den Oberflächen befinden, da die Wechselwirkungen zwischen Fe-Atomen und Einzelleerstellen-Graphen stärker sind als die zwischen Pt-Atomen und einzelne freie Stelle Graphen. Abbildung 3 veranschaulicht die Position von Pt- und Fe-Partikeln, die auf dem Graphenträger dispergiert sind, wie von Yang et al. vorgeschlagen. [101].
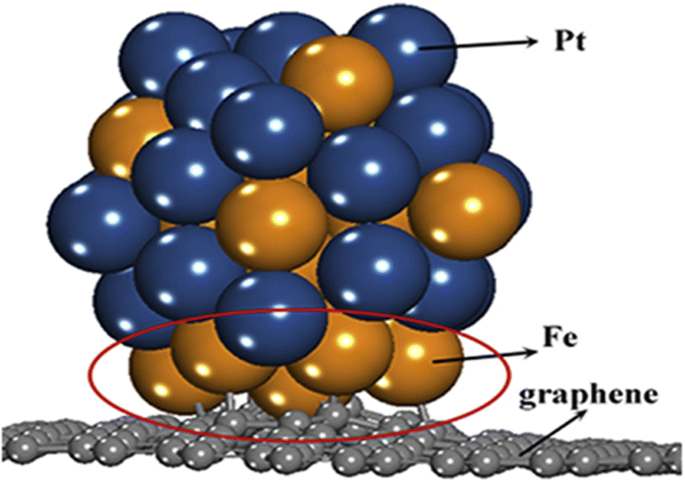
Die Position des PtFe-Katalysators auf einem Graphenträger illustriert von Yang et al. [101]
Leistung von Pt-basiertem Katalysator und Übergangsmetallkarbid
Als Anodenkatalysatoren können Übergangsmetallcarbide (TMC) mit hoher mechanischer und chemischer Korrosionsbeständigkeit, guter Beständigkeit gegen saure Umgebungen, Langzeitstabilität und hoher CO-Toleranz wirken [88,89,90, 102,103,104]. Darüber hinaus bieten TMCs gegenüber ihren Grundmetallen viele Vorteile hinsichtlich Aktivität, Selektivität und Giftresistenz, beispielsweise zeigt Wolframcarbid (WC) besondere Eigenschaften, wie gute elektrische Leitfähigkeit, Beständigkeit gegenüber saurer Umgebung, geringe Kosten und Toleranz gegenüber CO-Vergiftung bei der Methanol-Elektrooxidation [88, 105, 106].
Wanget al. [103] berichteten über die Synthese einer großen Oberfläche (256 m 2 g −1 ) Wolframcarbid-Mikrokugeln über eine einfache hydrothermale Methode. W2 C wurde als Hauptphase in der so synthetisierten Probe gefunden. Derzeit untersuchen Forscher das Potenzial von Pt auf WC als idealer Katalysator für DMFC [38, 88, 107, 108]. Christianet al. [106] kamen zu dem Schluss, dass sich TMCs in Bezug auf ihre Übergangsmetallelemente bei bestimmten chemischen und elektrochemischen Reaktionen, einschließlich der Oxidation von Wasserstoff, Kohlenmonoxid und Alkohol und der Reduktion von Sauerstoff, wie Edelmetalle wie Pt, Pd, Rh und Ru verhalten [109, 110]. In einer anderen Studie haben Liu et al. [107] präsentierten, dass Molybdäncarbide (Mo-Carbide) als Promotoren für Wolframcarbid wirken und die elektrokatalytische Aktivität in der DMFC erhöhen könnten. Ohne den Einbau von Pt-Metall ist die elektrokatalytische Aktivität von reinem WC gegenüber dem MOR für ein DMFC-System jedoch immer noch gering. Daher ist es sehr praktisch, der WC-Komponente eine kleine Menge Platinmetall zuzusetzen, um den Vorteil des synergetischen Effekts zwischen Pt und WC zu nutzen [91, 111, 112]. Inzwischen haben Hassan et al. [109] zeigten, dass die übliche Verunreinigung (CO-Spezies), die sich bei der Methanoloxidation bildet, eine starke Bindungsenergie an der Pt-Oberfläche besitzt; daher muss es oxidiert werden, damit es von den aktiven Pt-Zentren entfernt werden kann. Die Zugabe der WC-Komponente in den Pt/WC-Elektrokatalysator hat eine hohe CO-Toleranz für MOR gezeigt, was auf das Vorhandensein synergetischer Effekte zwischen dem Pt-Metall und WC als Trägerkomponente hinweist. Die Studie wurde auch von einem anderen Forscher durchgeführt, indem weniger Pt-Metall verwendet wurde, um die Kosten von Pt zu senken, während gleichzeitig eine gute elektrokatalytische Leistung beibehalten wurde.
Ansonsten ist die WC-Komponente aktiver für die Bildung einer Methoxygruppe (CH3 O-) als reines Pt [113, 114]. CV-Leistung von (Pt:Ru)4-WC/RGO zeigt hervorragende katalytische Leistung mit einer Stromdichte von 330,11 mA mg −1 Pt im Vergleich zu den anderen fünf Katalysatoren, was darauf hindeutet, dass der wie synthetisierte Elektrokatalysator eine ausgezeichnete katalytische Aktivität gegenüber dem MOR aufweist. Darüber hinaus erhöhte die Kombination von Ru und WC auf dem Pt-Katalysator die OH-Oberflächenmenge und ermöglichte die Oxidation des auf der Pt-Oberfläche adsorbierten CO bei niedrigeren Potentialen [39].
Leistung von Pt-basierten Katalysatoren und Übergangsmetallnitriden
Übergangsmetallnitrid (TMN) ist aufgrund seiner guten elektrischen Leitfähigkeit (metallisch), Härte, hohen elektrochemischen Stabilität und Korrosionsbeständigkeit unter Brennstoffzellen-Betriebsbedingungen ein idealer Kandidat als Pt-Katalysatorträger [115,116,117,118]. Es wurde über Übergangsmetallnitride wie CrsN, TiN und VN als Pt-Träger berichtet, die eine hohe katalytische Leistung und eine bessere Stabilität im Vergleich zu herkömmlichen Kohlenstoffträgern zeigten [112]. Alle Übergangsmetalle können Nitrid bilden, mit Ausnahme der zweiten und dritten Reihe der Metalle der Gruppe 8, 9 und 10 (Ru, Os, Rh, Ir, Pd und Pt). Das Verhalten und die strukturellen Eigenschaften von Übergangsmetallnitriden sind in der Literatur zu finden [92,93,94]. Xiaoet al. [112] stellten einen Titan-Kobaltnitrid-gestützten Pt-Elektrokatalysator her, der eine hervorragende Leistung und Stabilität gegenüber der Sauerstoffreduktionsreaktion (ORR) zeigte. Der Ti0.9 Co0,1 N-geträgerter Pt-Katalysator zeigte eine kleine Teilchengröße und eine gute Metalldispersion. Dieser hergestellte Elektrokatalysator behielt auch die elektrochemische Oberfläche (ECSA) von Pt bei und verbesserte die ECSA-Konservierung stark, wobei der frühe ECSA-Abfall nach 10.000 ADT-Zyklen nur um 35 % abnahm. Kobaltdotierung verbesserte die ORR-Aktivität und -Haltbarkeit erheblich. In der Zwischenzeit kann ein leistungsstarker und langlebiger Elektrokatalysator im DMFC-System unter Verwendung eines Pt(Ru)/TiN-Elektrokatalysators mit großer Oberfläche erhalten werden, der auch eine hohe elektrochemische Aktivität gegenüber MOR mit einer ∼ 52 %igen Verbesserung der katalytischen Aktivität und einer guten Stabilität/Haltbarkeit im Vergleich zeigte zu kommerziellem JM-Pt(Ru). Unterdessen erreichte die Einzelzellenleistung der DMFC eine um 56 % bessere maximale Leistungsdichte und zeigte eine hervorragende elektrochemische Stabilität für den CSG-Pt(Ru)/TiN-Elektrokatalysator [115].
Aktuelle Forschungen zu Pt-Nanopartikeln auf Titan-Eisen-Nitrid-Nanoröhren mit hohler und poröser Struktur und großer Oberfläche wurden von Li et al. [116] Es zeigte eine signifikante Zunahme der elektrokatalytischen Aktivität gegenüber dem MOR im sauren Zustand und hatte eine bessere Haltbarkeit. Die Gründe für diese Eigenschaften waren ihre experimentellen Daten, die bestätigten, dass die Zugabe von Fe die elektronische Struktur von Pt-Atomen abstimmen kann, was zur verstärkten Aktivität und Stabilität des Pt-Katalysators für den MOR beiträgt. In früheren Arbeiten von Xiao et al. [117], Pt/Ti0,8 Mo0,2 Der N-Katalysator zeigte eine poröse Struktur und eine große Oberfläche, kleine und gut dispergierte Pt-Nanopartikel. Dieses Katalysatorsystem bewahrte die intrinsische elektrochemische Stabilität der TiN-Nanostruktur und verbesserte bemerkenswert die MOR-Aktivität und -Haltbarkeit. Die derzeit verfügbaren Informationen zur elektrochemischen Stabilität von Wolframnitrid (WN) sind jedoch noch unzureichend [109].
MoxN (x = 1 oder 2) auf Ti-Substrat zeigte elektrochemische Stabilität in einem sauren Elektrolyten von 4,4 M H2 SO4 bis zu einem anodischen Potential von + 0,67 V (gegen SHE) über 50 wiederholte Zyklen [110]. Dieser Elektrokatalysator zeigte jedoch Oberflächenschäden wie Rissbildung und Zerbröckeln in den Bereichen mit hohem kathodischen (unter − 0.1 V vs. SHE) und anodischen (über + 0.67 V vs. SHE) Potential aufgrund von kathodischer bzw. anodischer Korrosion. Im Bereich des hohen anodischen Potentials oberhalb von + 0,67 V (gegenüber SHE) nahm die Sauerstoffzusammensetzung aufgrund der MoOx-Oxidbildung zu, was zur Deaktivierung führen könnte. Diese Ergebnisse zeigen, dass MoxN mit den im wässrigen Elektrolyten vorhandenen Sauerstoffspezies reagiert und oberhalb von + 0.67 V (gegen SHE) instabil ist. Mustafhaet al. [111] fanden heraus, dass auf TiN als Träger aufgebrachtes Pt eine Elektroaktivität für die Methanoloxidation zeigte, wobei ein hohes If/Ib-Verhältnis eine hohe CO-Resistenz im Voltammogramm mit einer Scanrate von 20 mV/s in 0.5 M CH . darstellt 3 OH + 0,5 M H2 SO4 als Elektrolyt. Als Ursache für die CO-Resistenz von Pt/TiN wurde der bifunktionelle Effekt zwischen Pt und TiN genannt. Darüber hinaus haben Ottakam Thotiyl et al. [91] erzielten gute Ergebnisse für einen Pt-beladenen TiN-Katalysator und zeigten eine sehr gute CO-Toleranz für die elektrochemische Oxidation von Methanol. Sie kamen zu dem Schluss, dass die besonderen Eigenschaften von TiN, die es als Pt-Träger für den MOR im alkalischen Medium geeignet machten, darin bestehen, dass es eine außergewöhnliche Stabilität, extreme Korrosionsbeständigkeit, gute elektronische Leitfähigkeit und ein starkes Adhäsionsverhalten aufweist. TiN-Trägerkatalysatoren sind vorteilhaft in Bezug auf Langzeitstabilität, Austauschstromdichte und stabile Ströme bei niedriger Überspannung. In den Experimenten wurden Platinbeladungen von 40 Gew.-% auf TiN verwendet.
In den letzten Jahren haben Liu et al. [118] successfully synthesized platinum on titanium nickel nitride decorated 3D carbon nanotubes which reduced graphene oxide (TiNiN/CNT-rGO) support by solvothermal process followed by nitriding process. Pt with small particle size is well-dispersed on TiNiN/CNT-rGO support. The 3D shape of CNT-rGO support gives a fast route for charge transfer and mass transfer as well as TiNiN NPs with good synergistic effect and the strong electronic coupling between different domains in TiNiN/CNT-rGO support. Thus, it greatly improved the catalytic activity of this catalyst. In another research, the non-carbon TiN nanotubes-supported Pt catalyst done by Xiao et al. [119] also displayed enhanced catalytic activity and durability toward MOR compared with the commercial Pt/C (E-TEK) catalyst.
Performance of Pt-Based Catalysts with Transition Metal Oxide
Pan et al. [92] reported the synthesis of platinum–antimony-doped tin oxide nanoparticles supported on carbon black (CB) as anode catalysts in DMFC, which exhibited better improvement in catalytic activity toward MOR compared to Pt-SnO2 /C or commercial Pt/C electrocatalyst. The enhancement in activity was attributed to the high electrical conductivity of Sb-doped SnO2 , which induced electronic effects with the Pt catalysts. Another work done by Abida et al. [93]described the preparation of Pt/TiO2 nanotube catalysts for methanol electrooxidation. The TiO2 nanotubes-supported Pt catalyst (Pt/TiO2 nanotubes) exhibited excellent catalytic activity toward MOR and had good CO tolerance. They also reported that the use of hydrogenotitanate nanotubes as a substrate for the Pt catalyst considerably improved the COads oxidation on Pt, but the MOR still occurred at high potential. Then, several years later, Wu et al. [94] synthesized Pt-C/TiO2 with MOR activity 1.6 higher than commercial Pt-C and the stability of Pt-C/TiO2 was also enhanced by 6.7 times compared to Pt-C. The excellent performance of this catalyst was a contribution of mesopores and partially coated carbon support. Zhou et al. [95] prepared hollow mesoporous tungsten trioxide microspheres (HMTTS) using the spray-drying method to yield Pt/HMTTS. The electrocatalyst exhibited excellent electrocatalytic activity and high stability toward MOR than Pt/C and Pt/WO3 electrocatalysts, which may be attributed to the well-ordered Pt particles (with an average size of 5 nm) on the HMTTS surface. Wuet al. [120] used polystyrene spheres as templates to obtain pore-arrayed WO3 (p-WO3 ). The Pt nanoparticles with an approximate size of 3.3 nm dispersed on pore-arrayed WO3 (Pt/p-WO3 ) exhibited high catalytic activity toward MOR.
Liet al. [121] used Sn-doped TiO2 -modified carbon-supported Pt (Pt/Ti0.9 Sn0.1 O2 –C) as an electrocatalyst for a DMFC system. The synthesized Pt/Ti0.9 Sn0.1 O2 –C electrocatalyst revealed high catalytic activity and CO tolerance toward MOR. The enhanced catalyst activity was due to the high content of OH groups on the Ti0.9 Sn0.1 O2 electrocatalyst sample and the strengthened metals and support interactions. In addition, Lv et al. [122] also reported in their work that the addition of TiO2 could not only facilitate CO removal and hinder CO formation on Pt surface during methanol oxidation, but it can also prevent the agglomeration and corrosion of Pt, which can be concluded from strong metal-supports interaction between TiO2 –C and Pt. Huang et al. [123] revealed that a TiO2 -coated carbon nanotube support for Pt electrocatalysts could be prepared via a one-step synthesis. Hao et al. [124] developed a new catalyst composed of Pt nanoparticles deposited on graphene with MoO3 . These catalysts exhibited high catalytic activity toward MOR and high resistance to CO species. However, the size of MoO3 must be tuned by controlling the metal oxide loading.
The selection of metal oxide such as MnO, RuO, CeO, SnO2 , MgO, and V2 O5 as additional component in electrocatalyst of Pt because of their low cost, good electrochemical properties, and have proton-electron intercalation properties [125]. From the catalytic activity aspect, it can be summarized that the addition of these metal oxides can enhance the electrocatalytic activity of DMFC and other fuel cells. The incorporation of these conducting metal oxides together with Pt catalyst could also facilitate the oxidation process of CO intermediate molecules. Hence, these types of metal have high potential to be used together with platinum as anode electrode.
Carbon support
To improve the utilization of the Pt catalysts, the carbon support is also another useful approach to be used together with Pt. Carbon materials are largely used as catalyst support because of its special properties such as relatively stable in both acid and basic electrolyte, good conductivity, and provide high surface area for dispersion of metal catalyst. It is believed that carbon materials have a strong effect that can influence the electrocatalysts properties such as metal particle size, morphology, metal dispersion, alloyed degree, and stability. Carbon supports can also affect the performance of supported catalysts in fuel cells, such as mass transport and catalyst layer electronic conductivity, electrochemical active area, and metal nanoparticle stability during the operation.
Currently, a great concern of the development in the nanotechnology field, especially carbon nanomaterials synthesis, is to create more stable and active supported catalysts. Support materials of nanoparticles are believed to be the most promising materials for catalytic activity in fuel cells, including the DMFC system. Pt has been traditionally used as nobel catalysts for many fuel cells application [126,127,128]. However, the high cost and low reserve are hindering commercialization of fuel cells and driving researchers to make the utmost of the catalyst. According to this problem, the major effort has been done toward nanoscaling of the catalyst nanoparticles to form more active sites per mass unit. The morphology, structure, and activity of the catalyst, and correspondingly the whole lifetime of a cell, thus strongly depend on the catalyst support [129]. Table 2 shows the preparation, physical properties, performance, and activity of Pt-based supported carbon done by groups of researchers. The details of Pt-based supported carbon will be performed in the following sections:“Graphene Support” to “Carbon Nanocoils”.
Graphene support
Graphene has many extraordinary properties; it exists as a two-dimensional carbon (2-D) form, which is called a crystalline allotrope, one-atom-thick planar flat sheet of sp2 tightly bonded carbon atoms with a thickness of 0.34 nm. Its carbon atoms are packed in a regular atomic-scale chicken wire (hexagonal) pattern [92, 119]. The theoretical specific surface area of graphene is 2630 m 2 g −1 , which is much larger than that of carbon black (typically less than 900 m 2 g −1 ) and carbon nanotubes (100 to 1000 m 2 g −1 ) and similar to that of activated carbon [130]. Graphene has high potential as a metal support [131, 132, 133] [33] due to its high surface area [134] for better catalyst/metal dispersion [135], high electrical conductivity [136], and good thermal properties [137, 138]. Moreover, the functionality of graphene support can be modified by changing it surface structure, and hence contribute to its potential applications, such as in fuel cells, energy storage, electrochemistry, supercapacitors, and batteries [138,139,140,141,142]. Figure 4 illustrates the preparation steps to obtain the graphene nanosheets (GNS), while Fig. 5 shows their TEM images [143]. It can be clearly observed that the thickness of the GO with many typical wrinkles obviously decreases compared to graphite. This can be explained by the presence of the rich oxygen-containing functional groups over the surface of GO [132]. Besides, both resulting GN-900 and GN-900-C contained of a large size of nanosheets structure, but the GN-900-C comprised more transparent than the GN-900.
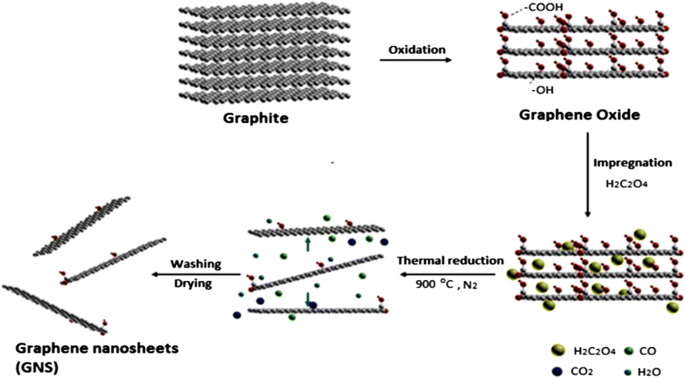
illustration of the preparation of graphite oxide to graphene nanosheets (GNS) by using oxalic acid [143]
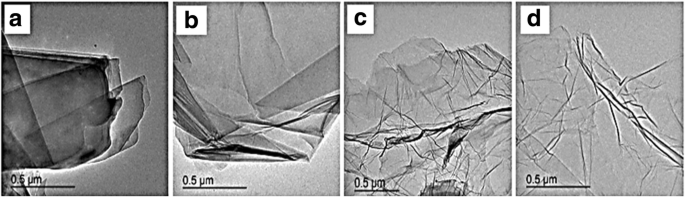
TEM images of graphite (a ), GO (b ), GN-900 (c ), and GN-900-C [143]
The discovery of graphene sheets began around 2000 by mechanical extracting process from 3D graphite source [133]. Graphene can be obtained by several synthesis methods such as hydrothermal [144], chemical reduction [143], chemical vapor deposition, and electrochemical. Ma et al. [145] enhanced the electrocatalytic activity of Pt nanoparticles by supporting the Pt nanoparticles on functionalized graphene for DMFC. Functionalized graphene was prepared by methyl viologen (MV) and Pt/MV–rGO electrocatalyst was synthesized by a facile wet chemical method. They also reported that the higher catalytic activity of Pt/MV–RGO was attributed to the synergetic effect between MV and rGO.
Meanwhile, Zhang et al. [146] modified the graphene support with graphene nanosheets through Hummer’s method, followed by polymerization of aniline (as nitrogen source). The TEM images for Pt/NCL-RGO and Pt/RGO electrocatalysts show that the aggregation between separated graphene sheets was decreased by nitrogen-doped carbon layer (NCL), leading to a better dispersion of the Pt catalyst on the graphene nanosheets support and better electroactivity and stability toward methanol electrooxidation (MOR). Presence of NCL successfully prevented the aggregation of graphene nanosheets as the Pt nanoparticles supporting material.
In 2011, Qiu et al. [135] successfully synthesized nanometer-sized Pt catalyst via sodium borohydride reduction method with an average particle size of only 4.6 nm. These Pt nanoparticles showed an even dispersion of Pt catalyst on graphene oxide support and very high electrocatalytic activity toward MOR by controlling the percent deposition of Pt loaded on the graphene. In another study conducted by Ojani et al. [147], for synthesized Pt-Co/graphene electrocatalyst, it was shown that graphene nanosheets improved the electrocatalytic behavior and long-term stability of the electrode. In addition, the Pt-Co/G/GC electrocatalyst showed great stability toward MOR. The catalytic performance toward MOR can also be improved by using cobalt core–platinum shell nanoparticles supported on surface functionalized graphene [148]. This enhanced catalytic activity could be attributed to the poly (diallyldimethylammonium chloride) (PDDA) that plays a crucial role for dispersion and stabilization of Co@Pt catalyst on graphene support. PDDA-functionalized graphene provided the higher electrochemical active surface area [149, 150]. Huang et al. [138] also studied a PtCo-graphene electrocatalyst with outstanding catalytic performance and high CO tolerance toward the MOR, which far outperformed Pt-graphene and PtCo-MWCNT electrocatalysts with the same ratio of Pt and carbon content. Figure 4 shows the formation of a graphene-PtCo catalyst prepared from a graphite source. Sharma et al. [57] synthesized Pt/reduced graphene oxide (Pt/RGO) electrocatalyst using a microwave-assisted polyol process, which sped up the reduction of GO and formation of Pt nanocrystals. They compared Pt/RGO to a commercial carbon support (Pt/C), which exhibited high CO tolerance, high electrochemically active surface area, and high electrocatalytic activity for the MOR. In a previous study, Zhao et al. [139] reported that the unique 3D-structured Pt/C/graphene aerogel (Pt/C/GA) exhibited greater stability toward MOR with no decrease in electrocatalytic activity. Moreover, the Pt/C/graphene aerogel also exhibited significantly higher stability to scavenge crossover methanol at high potential in an acidic solution compared with the commercial Pt/C electrocatalyst. At the initial catalytic stage, the Pt/C electrocatalyst lost approximately 40% after 1000 CV cycles. In contrast, the Pt/C/graphene aerogel only lost 16% of the initial catalytic activity. After 200 cycles of CV, the current density of Pt/C/graphene aerogel was much higher with a remarkably higher stability than that of Pt/C electrocatalyst. Meanwhile, Yan et al. [151] demonstrated highly active mesoporous graphene-like nanobowls supported Pt catalyst with high surface area of 1091 m 2 g −1 , high pore volume of 2.7 cm 3 g −1 , and average pore diameter of 9.8 nm obtained by applying a template synthesis method. In addition, the Pt/graphene bowls also achieved high performance toward MOR with a current density value of 2075 mA mgPt −1 , which was 2.87 times higher than that of commercial Pt/C (723 mA mgPt −1 ). The onset potential for the Pt/graphene bowls toward methanol electrooxidation was negatively shifted by approximately 160 mV compared with that to the latter and showed CO resistance. Figure 6 shows the proposed schematic for the formation of PtCo catalyst on reduced-GO (rGO) support [51]. It is described that the formation of graphene oxide nanosheets from oxidation of graphite powder leads to the increase in interlayer “d” spacing of stacked graphitic sheets from 0.34 to 0.78 nm due to the presence of various oxygen-containing functional groups. The oxygen-containing functional groups act as anchor sites for the well-dispersed Pt and PtCo nanoparticles on rGO sheets, and used for efficient electrooxidation of methanol.
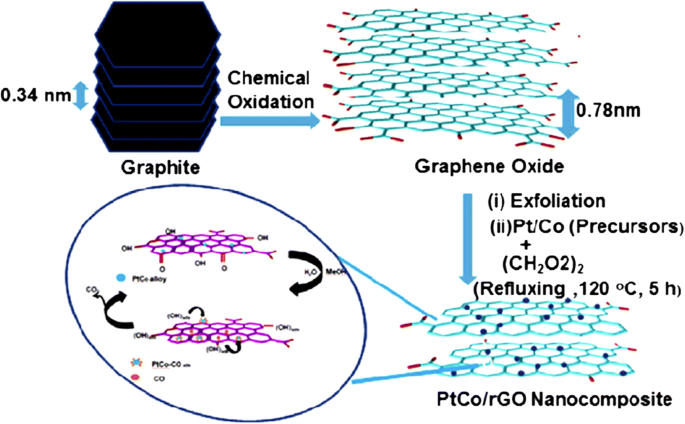
Illustrates the schematic formation of graphene supported Pt-Co catalyst [51]
We can conclude that the reduce graphene oxide (rGO), graphene, modified graphene as supporting material exhibited high electrocatalytic activity toward methanol electrooxidation process. A lot of studies have been reported related to the particle size distribution and size, morphologies, and catalytic activities of Pt and Pt alloys using graphene as supporting material, which showed great improvement in fuel cell performance as mentioned and discussed above. Thus, graphene support can be further studied for better fuel cell performance.
Multiwall Carbon Nanotube and Single-Wall Carbon Nanotube Support
Several years ago, Jha et al. [140] prepared multiwall carbon nanotube (MWCNTs) via chemical vapor deposition using an AB3 alloy hydride catalyst. Platinum-supported MWCNT (Pt/MWCNT) and platinum-ruthenium-supported MWCNT (Pt-Ru/MWCNT) electrocatalysts were prepared by chemical reduction. The performance of these electrodes was studied at different temperatures, and the results demonstrated a very high power density of 39.3 mW cm −2 at a current density of 130 mA cm −2 , which could be attributed to the dispersion and accessibility of the MWCNT support and Pt-Ru in the electrocatalyst mixture for the methanol oxidation reaction. This was also done by other researchers that using different catalyst supported MWCNT for DMFC system [152,153,154,155]. Meanwhile, Wu and Xu [156] compared MWCNT-supported Pt and single-wall carbon nanotube (SWCNT)-supported Pt. Figure 7 shows that the TEM images of Pt catalyst was deposited on MWNT and SWNT electrodes through the electrodeposition technique. The Pt particles in Pt-SWNT (Fig. 7b) looked closer contact with the network of entangled and branched bundles of SWNT support, and the shape is closer to highly exposed sphere. The benefits of the SWCNT support are due to its greater electrochemical surface-active area and easier charge transfer at the electrode/electrolyte interface because of the graphitic crystallinity structure, rich amount of oxygen-containing surface functional groups, and highly mesoporous and unique 3D-structure of SWNT. The electrodeposition technique carried out by them contributed to higher utilization and more uniform dispersion of Pt particles on its support.
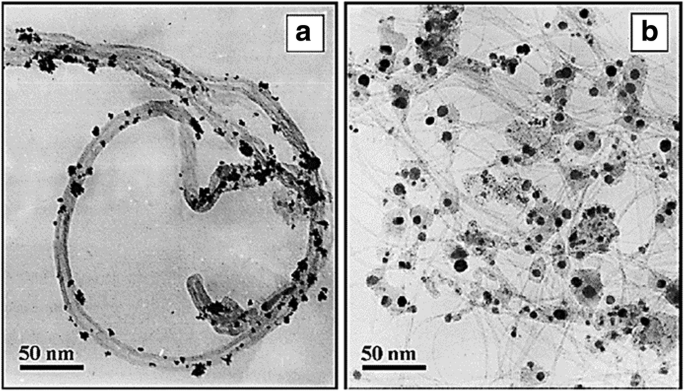
TEM images for the Pt on MWCNT(a ) and SWCNT (b ) [156]
Then, Wang et al. [157] reported the high performance of modified PtAu/MWCNT@TiO2 electrocatalyst prepared via deposition-UV-photoreduction for DMFC, which also exhibited high CO tolerance. Zhao et al. [126] studied 3D flower-like platinum-ruthenium (PtRu) and platinum-ruthenium-nickel (PtRuNi) alloy nanoparticle clusters on MWCNTs prepared via a three-step process, and the best ratios obtained from their experiments for the PtRu and PtRuNi alloys were 8:2 and 8:1:1, respectively. Another group, i.e., Zhao et al. [158], found a higher current density toward MOR and better activity for MWCNT-supported PtWC compared with Pt/C electrocatalyst. These results were attributed to the factors of the synergistic effect between the Pt catalyst and the WC component, high CO tolerance from the bifunctional effect of the Pt catalyst and the WC component, and strong interaction between metals and WC in the electrocatalyst composite.
As a summary, both of MWCNT and SWNT support in terms of structural, surface, and electrochemical properties have their own characteristics as supporting material that remarkably enhanced their performance in catalysis of methanol oxidation process. However, as a comparison, SWCNT possess a high degree of graphitization, highly mesoporous 3D structure, and contain more oxygen-containing functional groups at its surface sites. In relation with these properties, the SWCNT exhibits a higher electrochemically accessible surface area and faster charge transfer rate at the electrode/electrolyte interface.
Carbon Nanotube Support
Wen et al. [144] proposed that carbon nanotubes (CNTs) support could improve fuel cell performance; for example, Pt can be fixed to the inner wall and the outer wall of CNTs and may cause improvement in the electrocatalytic properties of platinum-CNTs. Yoshitake et al. [159] proposed that fuel cells using CNTs as the catalyst support produced larger current densities. The addition of binary or other components to the electrocatalysts for methanol electrooxidation overcomes the problems related to catalyst poisoning caused by CO during the reaction. Therefore, new electrocatalyst carbon supports, such as carbon nanotubes [160, 161], are being actively developed to significantly improve fuel cell performance. Kakati et al. [128] successfully synthesis the PtRu on CNT/SnO2 for anode catalyst DMFC via hydrothermal process. It has been found that the presence of SnO2 provide a high durability property for the catalyst and the presence of SnO2 in the district of Pt could supply oxygen-containing functional groups for the removal of CO intermediate molecules from the Pt surface sites during electrooxidation of methanol. Generally, the decomposition methanol occurs at Pt surface sites; meanwhile, the decomposition of water occurs at SnO2 surface sites to form oxygen-containing species which then react with CO intermediate molecules. However, as support material, the conductivity property of SnO2 still needs to be enhanced. Kakati et al. [128] also proposed the schematic diagram of the formation of PtRu on CNT/SnO2 composite as shows in Fig. 8, and FESEM images of CNT/SnO2 composite support (a and b) and PtRu/SnO2 /CNT composite electrocatalyst (c and d) in Fig. 9.
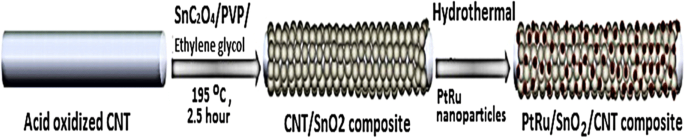
Illustrates the schematic diagram for the formation of PtRu/SnO2/CNT composite [128]
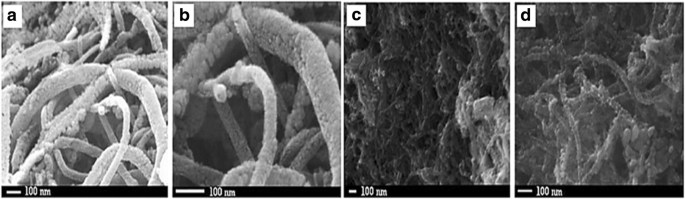
FESEM images of CNT/SnO2 composite support (a , b ) and PtRu/SnO2/CNT composite electrocatalyst (c , d )
Chien et al. [127] proposed that the high catalytic performance of Pt-Ru/CNT for MOR can be attributed to the presence of CNT as the carbon support material with several factors:(i) the as-synthesized Pt-Ru/CNT electrocatalyst owns the ideal nanosized particles and composition to increase catalytic activity, (ii) the presence of functional group on the CNT surface results in high hydrophilicity of CNT, which produces better electrochemical reaction on the electrode area, and (iii) the high electronic conductivity of the CNT support lowers the resistance in MOR. Jeng et al. [150] prepared Pt-Ru/CNT electrocatalyst via a modified polyol with a PtRu composition ratio of 1:1, exhibiting high catalytic activity toward MOR and better performance than that of commercial PtRu/C. Show et al. [162] reported that Pt catalyst with a size of less than 10 nm can be obtained by dispersing the Pt particles on a CNT surface using the in-liquid plasma method, and excellent performance was demonstrated by the electrical power achieving 108 mW cm −2 [162]. The in-liquid plasma method was also used by Matsuda et al. [163] that can applied to obtain nanometer-sized Pt catalyst on support material that remarkably enhanced the fuel cell performance.
To be concluded, high electric conductivity, large surface area, excellent chemical and electrochemical stabilities, quasi one-dimensional structure, and good morphology as the supporting materials are the key factors of carbon nanotubes (CNTs) in enhancing the DMFC performance. In addition, carbon support materials such as CNTs which contribute a large effect on metal distribution and size have also been proven to be an essential to the electrocatalysts to achieve high catalytic activity during methanol oxidation process.
Carbon Nanofiber Support
Steigerwalt et al. [164] reported the successful synthesis of PtRu alloy that was widely dispersed on a graphene carbon nanofiber (CNF) support as an electrocatalyst in DMFC. The catalytic activity was enhanced by ~ 50% relative to that recorded for an unsupported PtRu colloid anode electrocatalyst. Meanwhile, Wang et al. [152] reported that Pt/CNF nanocomposites obtained by the reduction of hexachloroplatinic acid (H2 PtCl6 ) precursor with formic acid (HCOOH) in aqueous solution containing electrospun CNFs at room temperature showed a higher current density than other prepared Pt/CNFs and was approximately 3.5 times greater than that of the E-TEK Pt/C electrocatalyst. Another research carried out by Giorgi et al. [153] described a CNF and bimetallic PtAu electrode with a single layer and both diffusive and catalytic functions using a decreased noble metal amount (approximately five times less) with a consequent large cost reduction. In addition, the bifunctional electrocatalytic properties were also active for the MOR on the PtAu nanoparticle catalysts [154]. Calderón et al. [155] reported PtRu/CNF prepared via reduction using sodium borohydride (NaBH4 ), methanol, and formate ions. This electrocatalyst synthesized by SFM was heat-treated (denoted as SFM TT), which improved its electrocatalytic activity during MOR. Later, Maiyalagan [165] reported that the addition of silicotungstic acid acted as a stabilizer for the PtRu particles on CNT support. The PtRu-supported CNT was prepared by microwave heating of an ethylene glycol (EG) solution of STA, H2 PtCl6 .6H2 O (as Pt precursor), and RuCl3 .xH2 O (as Ru precursor) with CNF suspended in the solution. The Pt and Ru precursors were loaded on CNF by conventional impregnation method. The results revealed that the PtRu nanoparticles are uniformly dispersed on carbon nanofiber support, with an average particle size of 3.9 nm enhanced the catalytic activity toward methanol electrooxidation. As a conclusion, the carbon nanotubes supporting material with high electronic conductivity and high surface area gives an advantage of better dispersion for the Pt or Pt alloys deposition. The higher the surface area of supporting material can reduce the agglomeration of metal particles on it, thus can produce better catalyst morphology for better fuel cell performance.
Mesoporous Carbon Support
Mesoporous carbon (MPC) support is another ideal candidate as an electrocatalyst support material in DMFC and fuel cell. Generally, mesoporous carbons are divided into two classes based on their structures which are ordered mesoporous carbons (OMCs), with highly ordered pore structure and uniform pore size, nonordered mesoporous carbons with irregular pores. Other than that, OPC can be produced by using high quality of SBA-15 silica and sucrose as carbon source template. To prepare the high quality of SBA-15 SBA-15 sample, triblock copolymer, EO20-PO70EO20 (Pluronic P123, BASF), as the surfactant and tetraethyl orthosilicate (TEOS, 98%, Acros) as the silica source are used, as reported by literature [166,167,168]. The synthesis of MPC starts from synthesis of SBA-15, followed by calcination process.
A well-dispersed and ultralow Pt catalyst (PtFe) supported on ordered mesoporous carbon (OMC) was prepared via a simple route and showed superior catalytic activity. The PtFe alloy with a size range of 3–5 nm was homogeneously dispersed on the CMS with a very high specific surface area of more than 1000 m 2 g −1 [169]. The incorporation of Fe was discussed in the previous section (“Performance of Various Types of Pt-Based Catalysts” section and “Performance of Pt-Based Alloys” section). The high specific surface area of mesoporous carbon support can be produced by carbonization process of a resorcinol-formaldehyde polymer with a cationic polyelectrolyte as a soft template [160]. The performance of Pt/MPC also related to the synthesis/preparation method as done by Kuppan and Selvam. Kuppan and Selvam [167] synthesized four type of Pt/mesoporous carbon by using different reducing agent which are NaBH4 , EG, hydrogen, and paraformaldehyde. From there, the Pt/mesoporous carbon synthesized using paraformaldehyde as reducing agent for showed highest current density. The highest in catalytic was attributed to the use of paraformaldehyde that gives the smallest Pt particle size (4.5 nm), and the highest ECSA (84 m 2 /g) belongs to Pt/mesoporous carbon.
Wang et al. [161] synthesized a Pt@WC/OMC electrocatalyst composite, in which the composite was platinized using a pulsed microwave-assisted polyol technique. The OMC produced in this synthesis exhibited high surface area property. The Pt@WC/OMC electrocatalyst also showed high activity, desirable stability, and CO tolerance toward MOR. In another work done by Zhang et al. [170], the ordered CMS had a unique hierarchical nanostructure (with a 3-D structure) with ordered large mesopores and macropores that facilitated the dispersion of Pt nanoparticles and rapid mass transport during the reactions.
To maximize the use of Pt particles, the support materials should have uniform dispersion, high utilization efficiency, and desirable activity and stability. Moreover, the good supporting materials must be suitable for surface chemistry, high loading of Pt dispersion, and some functional roles. Additionally, based on the previous studies as discussed above, the ordered mesoporous carbons with large pore sizes are highly desirable for fast mass transfer and, thus, enhance the catalytic activity especially in the reaction involve large reactants molecules.
Carbon Black
Carbon black (CB) is one of the commercial carbon support that has been used till now. There are many types of CB such as Vulcan XC-72, Black Pearl 2000, Denka Black, Shawinigan Black, Ketjen EC-300J, etc. [171, 172]. CB is commonly used as a carbon support material for electrocatalysts because it possesses high porosity properties, which make it suitable as a potential support material for the catalyst layer in PEMFCs and DMFCs as reported in provided literatures [173,174,175,176,177,178,179,180]. The comparison of the several carbon black support was reported by Wang et al. [181] who investigated the effect on DMFC performance using several types of carbon black such as Vulcan XC-72R, Ketjen Black EC 300J, and Black Pearls 2000 carbon black as additives/support for the Pt cathode catalyst. From the experiments, the results showed that Ketjen Black EC 300J was the most useful carbon support for increasing the electrochemical surface area and DMFC performance of the cathode catalyst.
Nowadays, CB is commercial carbon support for many fuel cell systems. Generally, it is used for the comparison with new or modified catalyst [125]. The following Table 3 summarizes the commercial carbon black and its properties for fuel cell application. There are so many modifications among carbon support materials and development of new carbon support for enhance fuel cell performance; however, commercial carbon black still is used in many fuel cell applications especially for the comparison with new or modified catalyst.
Carbon Nanocoils
Celorrio et al. [182] proposed carbon nanocoils (CNCs) as a PtRu support in their experiment, indicating that the electrocatalyst performance was strongly dependent on the synthesis method. CNC-supported electrocatalysts showed better electrochemical behavior than E-TEK electrocatalysts, and better electrocatalytic behaviors toward CO and methanol oxidation were achieved using CNC as a support material [182]. Sevilla et al. obtained highly graphitic CNCs from the catalytic graphitization of carbon spherules via the hydrothermal treatment of different saccharides which are sucrose, glucose, and starch [183]. They demonstrated that the high electrocatalytic activity of the CNCs is due to the combination of good electrical conductivity of their graphitic structure and high porosity property, which allows much less diffusional resistance of reactants/products. Two years later, Sevilla et al. [184] reported highly dispersed Pt nanoparticles on graphitic CNCs with diameters in the range of 3.0–3.3 nm and a very fine particle size distribution. The electrocatalyst possessed large active Pt surface area (up to 85 m 2 g −1 Pt), high catalytic activity toward MOR (up to 201 A g −1 Pt), and high resistance against oxidation, which was noticeably greater than that of the Pt/Vulcan electrocatalyst. Celorrio et al. [185] obtained Pt/CNC electrocatalysts via the impregnation method, which showed that a combination of Pt and CNCs facilitated the CO oxidation process.
Conductive Polymer Supports
Choi et al. [186] synthesized PtRu alloy nanoparticles with two types of conducting polymers, i.e., poly(N -vinyl carbazole) and poly(9-(4-vinyl-phenyl)carbazole), as the anode electrodes. Electrochemical and DMFC tests showed that these nanocomposite electrocatalysts were beneficial in a DMFC system, but their catalytic performance was still lower than that of a carbon supported electrode. Thus, they suggested that higher electrical conductivity of the polymer and lower catalyst loss are required in nanocomposite electrodes to achieve better performance in a DMFC. Choi et al. [171] and Kim et al. [172] prepared polyaniline (PANi) as a support material for PtRu catalyst in a DMFC system. PANi is a group of conductive polymers with high electronic conductivity and a methanol oxidation current similar to that of carbon-supported PtRu catalyst. Then, Kim et al. [172] conducted catalytic tests to compare PtRu/PANi support with PtRu/carbon support, showing that the enhanced catalytic activity of PtRu/PANi was due to (i) the high electrical conductivity of the polyaniline support, (ii) the increase of electrochemical surface area of the prepared electrocatalyst, and (iii) the higher ion diffusion behavior. In another study, Amani et al. [74] synthesized PtSn supported by C-PANI as an electrocatalyst with different Pt:Sn atomic ratios using the impregnation method. The PtSn/C-PANI electrocatalyst with a ratio of 30:70 showed outstanding performance in the methanol electrooxidation, and the current density was approximately 40% higher than PtRu/C and 50% higher than Pt/C-PANi. The CO tolerance and stability were improved compared to that of PtRu/C, and the methanol crossover was reduced. Yaldagard et al. [173] studied the electrocatalytic performance of Pt/PANi/WC/C electrocatalyst for methanol electrooxidation (MOR) and oxygen electro-reduction (ORR), and it exhibited higher MOR activity, high CO resistance, and improved stability compared to Pt/C electrocatalyst in the presence of methanol.
Wuet al. [174] presented polypyrrole nanowire networks (PPNNs) as the anodic microporous layers (MPLs) of passive DMFC. In passive DMFC system, the novel MPL achieved a 28.3% increase in the power density from 33.9 to 43.5 mW cm −2 compared with the conventional layer with a similar PtRu (1:1). The high performance was due to the presence of PPNNs, which expressively improved the catalyst utilization and mass transfer of methanol on the anode. Besides, Selvaraj and Alagar [175] prepared Pt-Ru nanoparticle-decorated polypyrrole/multiwalled carbon nanotubes (Ppy/CNT) via the in situ polymerization of Ppy on CNTs containing ammonium peroxydisulphate (NH4 )S2 O8 as an oxidizing agent at the temperature range of 0–5 °C, followed by deposition of Pt particles on PPy-CNT composite films via chemical reduction to produce Pt/PPy-CNT. It was found that the PtRu particles deposited on PPy–CNT composite films exhibited higher catalytic activity and stability toward MOR compared to Pt/PPy-CNT. So far, the investigation on polymer as supporting materials is not much as carbon support materials. From aspect as supporting materials, the performance of polymer support was not good/excellent as carbon support. Further studies are needed in the future for better electrocatalytic activity and DMFC performance.
Problems and Limitations of Using Pt for DMFC Systems
There are two major challenges in the development of new DMFC catalysts:(i) performance, including the catalytic activity, reliability, and durability; and (ii) catalyst cost reduction. Two major problems arise in DMFC when using pure Pt alone as the anode catalysts:(1) slower kinetics oxidation of methanol, even on some state-of-the-art anode catalysts, and methanol crossover through the membrane, which not only lowers cathode performance but also reduces fuel efficiency. To develop successful fuel cell technology, including DMFC technology, new catalysts must be investigated to improve the performance and reduce the cost. Reduction of the catalyst cost remains a major challenge. Currently, platinum is one of the most effective electrocatalysts for DMFC due to its high catalytic activity for methanol oxidation, but because it is a precious metal, platinum usage is challenging and limited [176, 177]. Therefore, many scientists have attempted to find materials that can behave like Pt catalysts. One problem with the MOR in DMFCs is that CO is produced as an intermediate reaction product when using Pt catalyst and has strong binding energy on platinum particles, poisoning the active sites of the platinum surface area [58]. Therefore, CO must be removed by oxidizing it from the Pt surface using another material with high resistance to CO poisoning. For example, Hwu et al. proposed Pt-modified WC catalyst that has remarkable resistance to CO poisoning [178]. On the other hand, they also suggested that CO tolerance originates from the lower CO desorption temperature on pure and Pt-modified WC compared to pure Pt.
There are many solutions that can be applied to reduce the cost of Pt, overcome or minimize the formation of CO species during methanol oxidation, and increase the kinetics of methanol oxidation, such as alloying with other metals or transition metals, the incorporation of metals, metal nitrides, and metal oxides and the use of carbon supports as discussed in this paper. However, to overcome this problem, we need to understand the formation of CO on Pt sites particle, and understanding of the mechanism of the anode reaction in DMFCs. Unfortunately, it has limited amount of mechanistic insight to be studied, because this reactions involve complex mechanism path with many possible intermediate molecules and also competing reaction pathways [179]. For Pt catalytic mechanism, it has been suggested by a direct reaction path. Unfortunately, the use of Pt on other metals has limited mechanistic information available. Figure 10 represents the reaction path for methanol electrooxidation and their possible intermediates molecules formed during the process. Black arrows show direct path, while green arrows show the indirect mechanism for CO2 formation as a final product. In the direct mechanism, the reaction path does not involve a CO intermediate, and CO2 is formed directly from methanol. In contrast, indirect mechanism forming a CO intermediate molecule and subsequently it is oxidized to CO2 product. Notably, CO is the most stable molecule of all the intermediates on Pt during MOR. The stability of CO causes it to be a main reason for the extensive CO poisoning problem that is often found on Pt catalyst.
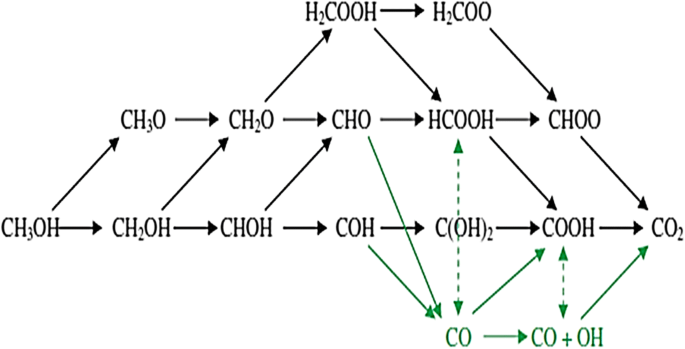
Schematic of the reaction paths and possible intermediates molecules considered in methanol electrooxidation [237]
First step in the mechanism of methanol decomposition reaction on Pt is the activation of methanol molecule. It can take place via hydrogen abstraction from either the carbon or the oxygen atoms. Further step, hydrogen abstraction creates formaldehyde (CH2 O) or hydroxymethylene (CHOH), followed by formyl (CHO) or COH. In the direct mechanism, instead of stripping off the final hydrogen from CHO or COH molecule to CO, a water molecule will release a proton/electron pair and resulting to OH species that can further bind with the carbonaceous species to form dihydroxycarbene (C(OH)2 ) or formic acid (HCOOH). This step is called hydroxyl addition process. The next step is followed by dehydrogenation to form either formate (HCOO) or carboxyl (COOH) molecule, with subsequent dehydrogenation to form CO2 as the final product of reaction. In addition, an alternative direct mechanism involve the stripping of a proton/electron pair from water and addition of the resulting hydroxyl to CH2 O, subsequently to H2 COOH, which then undergoes dehydrogenation to form HCOOH or dioxymethylene (Hs COO). The Hs COO molecule can then undergoes dehydrogenation to HCOO and finally to CO2 . Besides, in the indirect mechanism, CHO or COH species are directly dehydrogenated to CO. Water is dissociated separately on the surface to form OH, and the two surface species react together to form CO2 gas in a way similar to the water-gas-shift reaction [187]. This indirect mechanism occurs because less energy is required to form CO than CO2 . Strong adsorbed CO intermediate form on the Pt surface sites revealed a major problem at the anode site of DMFC. Formation of this intermediate species can cause deactivation Pt catalyst. Furthermore, the rate of kinetic methanol oxidation for DMFC is slower. Therefore, to increase the resistance of Pt catalyst to CO poisoning on the electrodes, Pt alloy or hybrids, such as PtRu, PtSn, PtMO, PtPb, PtFe, PtCo, PtNi, PtRuOs, PtRuMo, PtRuSn, PtRuNi, etc. (as mentioned and discussed in “Performance of various types of Pt-based catalysts” section), are usually employed as electrocatalyst materials on DMFC anodes. Addition/incorporation of these alloys to Pt can prevent the adoption of CO on Pt surface by decreasing the oxidation overpotential of the anode [84].
Conclusion and Prospects
Great progress has been made in recent years in the development and optimization of new catalysts using Pt-based catalysts and carbon and conductive polymer supports for DMFC anode catalyst. Some new carbon materials, such as nano- or mesostructured carbons, have been demonstrated as highly potential catalyst support materials, although their applications face challenges in terms of synthesis, metal loading, and electrode preparation. The combination of platinum as the best metal catalyst for DMFC and an excellent carbon support could produce breakthroughs in the investigation of a new DMFC anode catalyst in the future. Since platinum is an expensive metal, it is necessary to reduce the amount of Pt used in the electrocatalyst. Therefore, this paper presented more than 100 studies on the electrocatalytic activity and performance related to Pt-based electrocatalysts and various carbon and conductive polymer supports. The main problems related to the platinum electrocatalyst, such as carbon monoxide formation during the methanol oxidation reaction and the poor kinetics of methanol oxidation, could be overcome using additional materials and various supports, as reported in the research presented in this paper.
Many studies conducted in the recent years to reduce the loading amount of Pt catalyst and to increase the percentage utilization efficiency, and hence, enhance the electrocatalytic activity of Pt toward the oxygen reduction reaction (ORR) and methanol electrooxidation reaction (MOR), were discussed in this paper. Pt has been alloyed with many transition metals such as Fe, Co, Ni, Ir, Ru, Rh, and Pd, resulting in higher catalytic activity for the DMFC system. The incorporation of these materials also resulted in good dispersion on the carbon and polymer supports, which showed higher performance in the DMFC test compared to the use of Pt metal alone. Various carbon support sources, namely activated carbon (AC), carbon black (CB), multiwall carbon nanotubes (MWCNTs), carbon nanofibers (CNFs), carbon nanotubes (CNTs), graphene, and conductive polymer supports, have been used with Pt-based catalysts to improve their catalytic performance. Additionally, Pt-based alloy catalysts have been designed as hollow mesoporous PtNi, nanowire PtRu, and nanodendritic PtRh, which showed improved electrocatalytic activity and superior electrocatalytic performance. Meanwhile, 3-D Pt/C/graphene aerogel demonstrated enhanced stability toward methanol electrooxidation. The work performed by researchers showed that the electrocatalytic activities of nanoparticles Pt alloy catalysts depend on several factors such as the synthesis method, condition of experiments (such as temperature and pH), alloy composition/ratio, precursors, and thermal treatment. For the future study, it should be extended to the optimization of the geometry and structure of previous studies that revealed active Pt alloys can increase their electrocatalytic activity and stability and the application of support materials for fuel cell applications. For example, current research that have been done by Liu et al. 2017 [188] shows the excellent performance of platinum. From theoretical calculations, it revealed that the main effective sites on platinum single atom electrocatalysts are single-pyridinic-nitrogen-atom-anchored single-platinum-atom centers, which ascribed to the tolerant CO in MOR. They also suggested that carbon black supported used together with Pt single atom is effective in cost, efficient, and durable electrocatalyst for fuel cell application. According to the above study, herein, we can conclude that the modification on structure and morphology of precious metal such as platinum could also remarkably increase the performance of electrocatalyst, but in the same time can reduce the overall cost of fuel cell for commercialization.
To improve the morphologies of Pt and Pt alloys, carbon support material also need further study. Nanoporous metals become an interesting part of catalyst to be studied for fuel cell application. It is determined very suitable for fuel cell catalysts because they possess high surface area, three-dimensional (3D) network structures with adjustable ligament/pore sizes suitable for mass transport, and electron conduction. Around 2017, Li et al. successfully carried out modification on Pt-Pd-Au trimetallic surface as cathode for oxygen reduction reaction [189]. The surface evolution of 3-D Pt-Pd-Au trimetallic greatly enhanced the ORR activity and highly stable as ORR catalyst. The modification of PtNi alloy also done by Li et al. 2016 [190] showed ultrafine jagged platinum nanowire with highly large ECSA that exhibits enhanced mass activity of ~ 50 times higher than state-of-the-art commercial Pt/C catalyst, while Bu et al. 2016 [191] reported highly uniform PtPb/Pt core/shell nanoplate with biaxially strain extremely active, stable for anodic oxidation reactions, and great performance compared to commercial Pt/C in both methanol oxidation reaction (MOR) and ethanol oxidation reaction (EOR). Since the nanostructured platinum becomes an efficient catalyst for fuel cells as well as various industrial chemical reactions. Thus, these modifications on surface of Pt particles electrocatalysts could also to be applied in MOR for future DMFC.
On the other hand, to reduce the consumption of the Pt catalysts, the modification of the carbon support is also another useful way. This not only improves the transport capacity of protons but also reduces the usage of Nafion, which can cut the cost of the fuel cell. Moreover, with regards to the carbon support for the ORR catalysis, the hydrophobic carbon support material is required to allow water (product) to be quickly removed from the catalyst surface sites, and oxygen (reactant) to access the active sites. In contrast, the MOR catalysis requires a certain degree of hydrophilic carbon support. It can be achieved by the modification of the carbon support materials. By combination of modified carbon support materials and development of new carbon support with Pt metal catalyst, it is possible to get an ideal electrocatalysts for direct methanol fuel cell technology. Combination of Pt metal with varied carbon supports with different specific surface areas, structures, pore sizes, electronic properties, and morphologies could be great catalyst to be studied for future DMFC.
Carbon support also influence the overall performance for DMFC. Vulcan XC-72R, which is a commercial carbon support, has a large surface area, appropriate particle size, and good electrical conductivity for good support. However, in the process of depositing metal particle on these support with loading of 40% or more, the particle size of metal increased quickly, which is a disadvantage for DMFC, because a higher metal loading is used to give a better performance. In addition, multiwalled carbon nanotubes (MWCNTs) and carbon nanofibers (CNFs) with relatively smaller surface area, large diameter, and high aspect ratio could be very difficult to deposit a catalyst with high loading metal (40% and more). Therefore, modification of MWCNTs and CNFs support must be done to improve its surface area, surface functional groups, and reduce the wall thickness to achieve outstanding performance for direct methanol fuel cell even though high loading metal catalyst is consumed. As well, a great and important part to be further studied in DMFC system is about the anode and cathode catalyst preparation approaches.
Abkürzungen
- CB:
-
Carbon black
- CH3 O:
-
methoxy group
- CNC:
-
Carbon nano cage
- CNF:
-
Carbon nano fiber
- CNT:
-
Carbon nano tube
- Co:
-
Cobalt
- Co:
-
Cobalt
- CO:
-
Monoxide molecules
- CO2 :
-
Carbon dioxide
- DMFC:
-
Direct methanol fuel cell
- FC:
-
Fuel cell
- Fe:
-
Iron
- MOR:
-
Methanol oxidation reaction
- MPC:
-
Mesoporous carbon
- MWCNT:
-
Multi wall carbon nanotube
- Ni:
-
Nickel
- OMC:
-
Ordered mesoporous carbon
- ORR:
-
Oxygen reduction reaction
- PANi:
-
Polyaniline
- PEMFC:
-
Proton exchange membrane fuel cell
- Ppy:
-
Polypyrrole
- Pt:
-
Platinum
- Pt/MWCNT:
-
Platinum-supported MWCNT
- Pt-Ru/MWCNT:
-
Platinum-ruthenium-supported MWCNT
- Rh:
-
Rhodium
- Ru:
-
Ruthenium
- Sn:
-
Sternum
- SOFC:
-
Solid oxide fuel cell
- SWCNT:
-
Single-wall carbon nanotube
- TMN:
-
Transition metal nitride
Nanomaterialien
- Multifunktionale Goldnanopartikel für verbesserte diagnostische und therapeutische Anwendungen:Eine Übersicht
- Fortschritte und Herausforderungen fluoreszierender Nanomaterialien für die Synthese und biomedizinische Anwendungen
- Graphen- und Polymerverbundstoffe für Superkondensatoranwendungen:ein Rückblick
- Herstellung und Charakterisierung eines neuen anodischen Tio2-Kohlenstoff-Nanofaser-Verbundkatalysators für eine Direkt-Methanol-Brennstoffzelle mittels Elektrospinnverfahren
- Verbesserte Leistung eines neuartigen anodischen PdAu/VGCNF-Katalysators für die Elektrooxidation in einer Glycerin-Brennstoffzelle
- Bewertung von Graphen/WO3- und Graphen/CeO x -Strukturen als Elektroden für Superkondensatoranwendungen
- Neuartige anodische Katalysatorunterstützung für Direktmethanol-Brennstoffzelle:Charakterisierungen und Einzelzellleistung
- Biomedizinische Anwendungen für Gold-Nanocluster:Jüngste Entwicklungen und Zukunftsperspektiven
- Übersicht:Poröse Metallfilter und Membranen für die Öl-Wasser-Trennung
- Solvay bringt Hochleistungs-Kohlefaserband für Offshore-Öl- und Gasanwendungen auf den Markt